10 2.4 Earth’s First 2 Billion Years
Setting the Stage
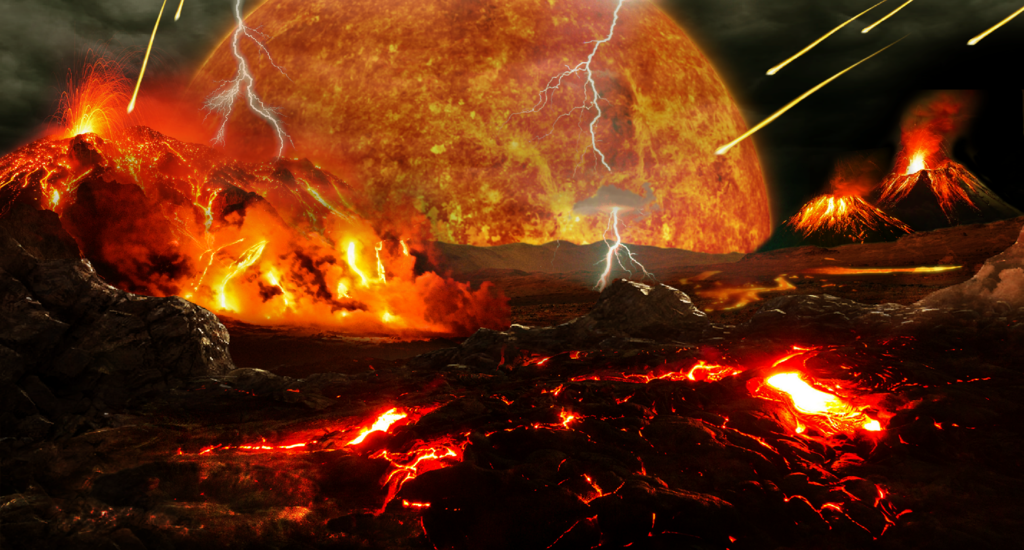
If you were to get into a time machine and visit Earth shortly after it formed (around 4.6 billion years ago), you would probably regret it. Large patches of Earth’s surface would still be molten, which would make landing your time machine very dangerous indeed.
If you happened to have one of the newer time-machine models with hovering capabilities and heat shields, you would still face the inconvenience of having nothing to breathe but a tenuous wisp of hydrogen and helium gas, and depending on how much volcanic activity was going on, volcanic gases such as water vapour and carbon dioxide. Some ammonia and methane might be thrown in just to make it interesting, but there would be no oxygen.
Assuming you had the foresight to purchase the artificial atmosphere upgrade for your time machine, it would all be for naught if you materialized just in time to see an asteroid—or worse yet, another planet—bearing down on your position. The moral of the story is that early Earth was a nasty place, and a time machine purchase is not something to take lightly.
How Earth Developed Interior Layers
How Do We Know the Composition of Earth’s Layers?
In the previous section, you read about how terrestrial planets such as Earth have a rocky mantle and a metallic core. Earth’s core is mostly a mixture of iron (Fe) and nickel (Ni). The mantle consists mostly of silicate minerals high in iron and magnesium (Mg). Silicate minerals are those having silica (Si) and oxygen (O) defining their basic structure and properties. If you think back to how elements are made, you will realize these elements are old friends we’ve already met.
We can’t observe the core directly, nor much of the mantle. Nevertheless, we can be confident in this assessment of Earth’s composition because we know Earth’s density.
Need a refresher on density?
Density refers to how heavy an object is for its size, and it’s helpful because it tells us about the materials that make up an object.
You already have an intuitive sense of what density means. Imagine you received a package in the mail. You ordered a fluffy sweater and some books, so either could be in the package. You would know immediately which was in the package just by lifting it. If it felt heavy you’d know it contained books. Books have a greater density than a fluffy sweater.
Similarly, density tells us something about the materials that make up a planet. Earth’s density reflects the fact that it has a metallic core and a rocky mantle. The iron making up Earth’s core has density of 8.0 g/cm3. A density of 3.25 g/cm3 is a good estimate for mantle rocks. Together these components give Earth a density of 5.5 g/cm3.
Density is used to approximate the composition of other planets in our solar system, as well as the compositions of newly discovered planets in other solar systems.
We also know about Earth’s composition because we have samples of broken up terrestrial planets and asteroids to look at. These are in the form of meteorites—mm-sized to meter-sized fragments originating in the asteroid belt. Meteorites consisting entirely of iron (Figure 2.13) are thought to represent core fragments from shattered asteroids and terrestrial planets. Stony meteorites represent the rocky exteriors.
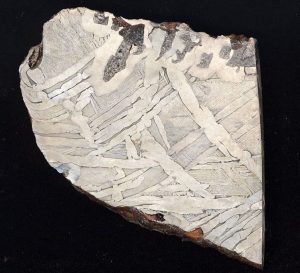
Differentiation: Earth Unmixes Itself
Today Earth has a layered interior, but early in its history it was a disorganized mixture of whatever metallic and silicate minerals it grabbed up in its orbit around the young sun. The decay of radioactive elements, plus objects smashing into Earth heated it up enough to melt the iron within. Dense melted iron sank to the centre of out planet, forming its core, and the remaining silicate material formed the mantle. The process of separating melted iron and silicate minerals into layers within Earth is called differentiation.
This makes it sound like a foregone conclusion that the melted iron would sink to Earth’s centre, but think about a chocolate chip cookie for a minute, and imagine what would happen if you put it in the microwave long enough to melt the chips. Would you have a layer of chocolate at the base of the cookie, and a layer of chocolate-free cookie above? No, because some of the chocolate would remain trapped in pockets of cookie dough. Early Earth was an analogous scenario, except the melted iron was trapped in pockets of silicate mantle rocks.
For a long time, scientists thought that the solution to this problem was to melt the entire Earth—silicate minerals and metal—so the metal could escape. More recently, a study demonstrated that when silicate rocks with pockets of iron melt were deformed in the lab (as we would expect early Earth to be deformed periodically by space objects slamming into it), it opened channels between blobs of melted iron, permitting the iron to flow (Figure 2.14). This would have allowed differentiation to proceed with far less heat involved.
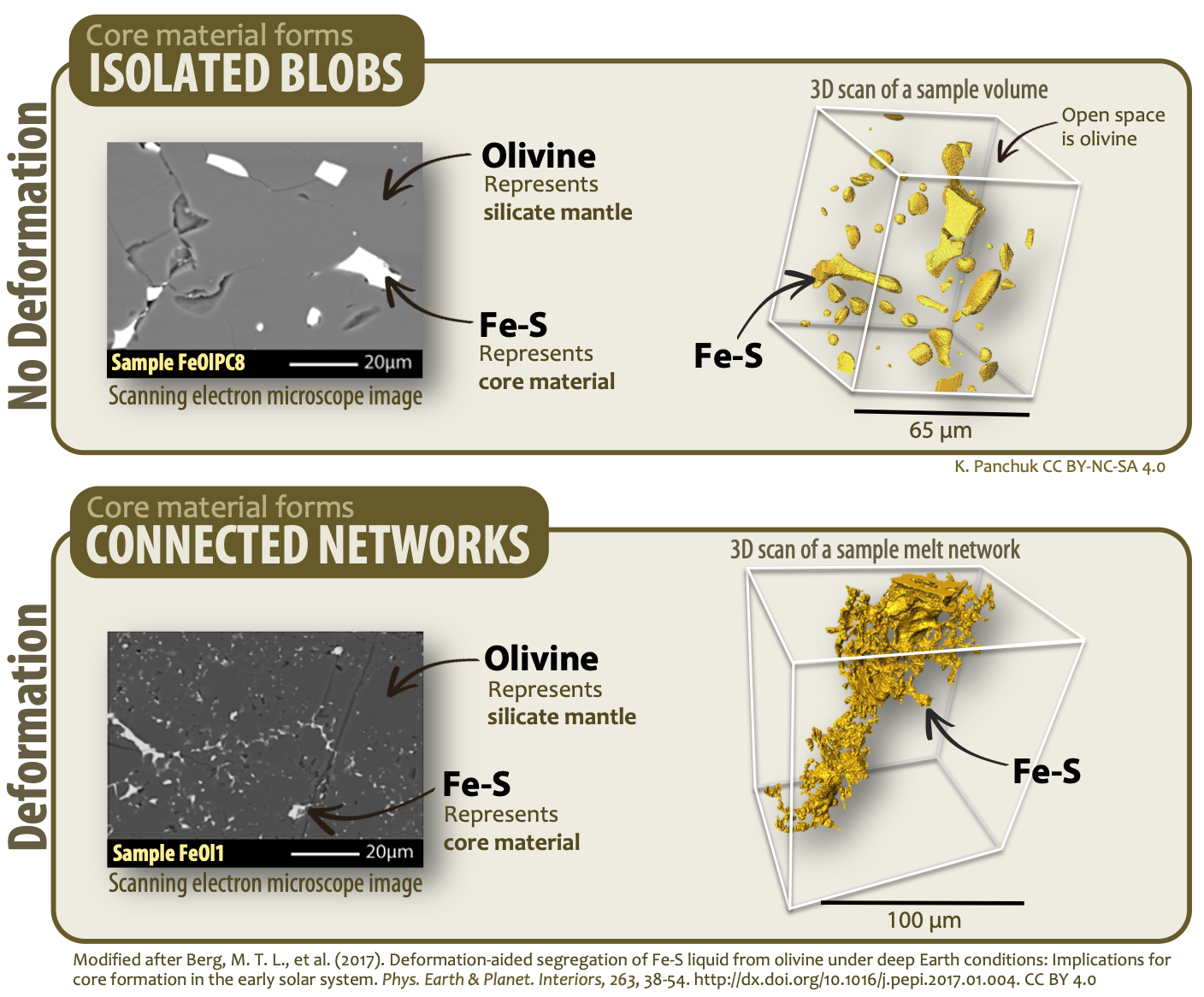
Capturing A Moment in Time
A Catastrophic Disruption
Although Earth had swept up a substantial amount of the material in its orbit as it was accreting, unrest within the solar system caused by changes in the orbits of Saturn and Jupiter was still sending many large objects on cataclysmic collision courses with Earth. The energy from these collisions repeatedly melted and even vaporized minerals in the crust, and blasted gases out of Earth’s atmosphere.
Very old scars from these collisions are still detectable, although we have to look carefully to see them. For example, the oldest impact site discovered is the 3 billion year old Maniitsoq “crater” in west Greenland, although there is no crater to see. What is visible are rocks that were 20 km to 25 km below Earth’s surface at the time of the impact, but which nevertheless display evidence of deformation that could only be produced by intense, sudden shock.
The evidence of the very worst collision that Earth experienced is not subtle at all. In fact, you’ve probably looked directly at it thousands of times already, perhaps without realizing what it is. That collision happened not long after Earth formed, with a planet named Theia, which was approximately the size of Mars (Figure 2.15).
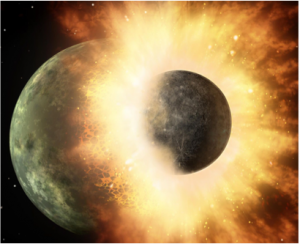
When Theia slammed into Earth, Theia’s metal core merged with Earth’s core. Debris from the outer silicate layers was cast into space, forming a ring of rubble around Earth. The material within the ring coalesced into a new body in orbit around Earth, giving us our moon. Remarkably, the debris may have coalesced in 10 years or less! This scenario for the formation of the moon is called the giant impact hypothesis.
Evidence for the giant impact hypothesis comes from chemical fingerprints in rocks on Earth and rocks on the moon. The moon has less iron that we would expect (thanks to Theia donating its core to us), and more elements that condense under high temperatures (thanks to having condensed from material vapourized into space).
Prior to the chemical evidence for the giant impact hypotheses, other moon-forming hypotheses under consideration included Earth grabbing an already-formed moon as it tried to whiz by us, and a very gloopy melted Earth spinning fast enough to throw off a glob of itself into space.
Today’s Atmosphere Took a Long Time to Develop
Earth’s first experiment with having an atmosphere did not succeed. It started out with a thin veil of hydrogen and helium gases that came with the material it accreted. But hydrogen and helium are very light gases, and they bled off into space.
Earth’s second experiment with having an atmosphere went much better. Volcanic eruptions built up the atmosphere by releasing gases. The most common volcanic gases are water vapour and carbon dioxide (CO2), but volcanoes release a wide variety of gases. Other important contributions include sulphur dioxide (SO2), carbon monoxide (CO), hydrogen sulphide (H2S), hydrogen gas, and methane (CH4). Meteorites and comets also brought substantial amounts of water and nitrogen to Earth. It’s not clear what the exact composition of the atmosphere was after Earth’s second experiment, but carbon dioxide, water vapour, and nitrogen were likely the three most abundant components.
One thing we can say for sure about Earth’s second experiment is that there was effectively no free oxygen (O2, the form of oxygen that we breathe) in the atmosphere. We know this in part because prior to 2 billion years ago, there were no rocks stained red from oxidized iron minerals. Iron minerals were present, but not in oxidized form. At that time, O2 was produced in the atmosphere when the sun’s ultraviolet rays split water molecules apart, but chemical reactions removed the oxygen as quickly as it was produced.
It wasn’t until well into Earth’s third experiment—life—that the atmosphere became oxygenated. Photosynthetic organisms used the abundant CO2 in the atmosphere to manufacture their food, and released O2 as a by-product. At first all of the oxygen was consumed by chemical reactions as before, but eventually the organisms released so much O2 that it overwhelmed the chemical reactions. Oxygen began to accumulate in the atmosphere, although present levels of 21% oxygen didn’t occur until about 350 million years ago. Today the part of our atmosphere that isn’t oxygen consists largely of nitrogen (78%).
The oxygen-rich atmosphere on our planet is life’s signature. If geologic processes were the only ones controlling our atmosphere, it would consist mostly of carbon dioxide, like the atmosphere of Venus. It’s an interesting notion (or a disconcerting one, depending on your point of view) that for the last 2 billion years the light reflected from our planet has been beaming a bar code out to the universe, similar to the ones in Figure 2.4, except ours says “oxygen.” For 2 billion years, our planet has been sending out a signal that could cause an observer from another world to say, “That’s odd… I wonder what’s going on over there.”
Earth: No Longer a Smashing Time
References
Berg, M. T. L., et al., (2017). Deformation-aided segregation of Fe-S liquid from olivine under deep Earth conditions: Implications for core formation in the early solar system. Physics of Earth and Planetary Interiors, 263, 38-54. http://dx.doi.org/10.1016/j.pepi.2017.01.004. CC BY 4.0. Open as a PDF.