Unit 8.1: Innate Immunity
Outline
- Chemical and Enzymatic Mediators Found in Body Fluids
- Antimicrobial Peptides
- Plasma Protein Mediators
Learning Objectives
After reading the following, you should be able to:
- Define innate immunity and adaptive immunity.
- Differentiate between the first and second lines of defense.
- Differentiate between physical versus chemical factors in the first line of defense.
- Describe the role of the normal flora in the innate immunity.
- Describe the role of the following components of the second line of defense:
- Immune cells (including Mast cells, Natural killer cells and Phagocytes)
- Phagocytosis (identify which cells are phagocytes and be able to describe the stages)
- Inflammation (signs, functions and stages)
- Fever (mechanism and advantages to host)
- Antimicrobial substances
- Describe and give an example of cytokines important to the innate immune system.
- Describe how pathogens can resist death by phagocytosis.
- Describe how bacteria can evade complement system.
- Describe why interferons can be used as a drug and describe the issues that come with using interferons as a drug.
Despite relatively constant exposure to pathogenic microbes in the environment, humans do not generally suffer from constant infection or disease. Under most circumstances, the body is able to defend itself from the threat of infection thanks to a complex immune system designed to repel, kill, and expel disease-causing invaders. Immunity as a whole can be described as two interrelated parts: nonspecific innate immunity, which is the subject of this section, and specific adaptive host defenses, which are discussed in the next section.
The nonspecific innate immune response provides an initial defense that can often prevent infections from gaining a solid foothold in the body. These defenses are described as nonspecific because they do not target any specific pathogen; rather, they defend against a wide range of potential pathogens. They are called innate because they are built-in mechanisms of the human organism. Unlike the specific adaptive defenses, they are not acquired over time and they have no “memory” (they do not improve after repeated exposures to specific pathogens).
Broadly speaking, nonspecific innate defenses provide an immediate (or very rapid) response against potential pathogens. The innate immune response can roughly be separated into two divisions, the first line and second line of defense. The first line of defense involves preventing pathogens from gaining access to the body and includes features such as the skin and mucous membranes. The second line of defense comes into play when pathogens are able to bypass these initial barriers and includes chemical and cellular responses. However, these responses are neither perfect nor impenetrable. They can be circumvented by pathogens on occasion, and sometimes they can even cause damage to the body, contributing to the signs and symptoms of infection (Figure 8.1).
Physical Defenses: Nonspecific innate immunity can be characterized as a multifaceted system of defenses that targets invading pathogens in a nonspecific manner. In this section, we have divided the numerous defenses that make up this system into three categories: physical defenses (first-line), chemical defenses (first-line or second-line), and cellular defenses (second-line). However, it is important to keep in mind that these defenses do not function independently, and the categories often overlap. Table 8.1 provides an overview of the nonspecific defenses discussed in this section.
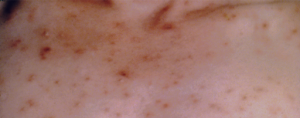
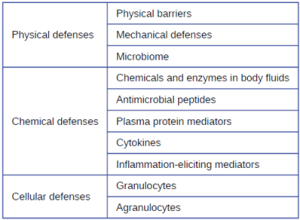
Physical defenses provide the body’s most basic form of nonspecific defense. They include physical barriers to microbes, such as the skin and mucous membranes, as well as mechanical defenses that physically remove microbes and debris from areas of the body where they might cause harm or infection. In addition, the microbiome provides a measure of physical protection against disease, as microbes of the normal microbiota compete with pathogens for nutrients and cellular binding sites necessary to cause infection.
1. Physical Barriers: Physical barriers play an important role in preventing microbes from reaching tissues that are susceptible to infection.
At the cellular level, barriers consist of cells that are tightly joined to prevent invaders from crossing through to deeper tissue. For example, the endothelial cells that line blood vessels have very tight cell-to-cell junctions, blocking microbes from gaining access to the bloodstream. Cell junctions are generally composed of cell membrane proteins that may connect with the extracellular matrix or with complementary proteins from neighboring cells. Tissues in various parts of the body have different types of cell junctions. These include tight junctions, desmosomes, and gap junctions, as illustrated in Figure 8.2. Invading microorganisms may attempt to break down these substances chemically, using enzymes such as proteases that can cause structural damage to create a point of entry for pathogens.
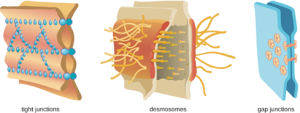
a. The Skin Barrier: One of the body’s most important physical barriers is the skin barrier, which is composed of three layers of closely packed cells. The thin upper layer is called the epidermis. A second, thicker layer, called the dermis, contains hair follicles, sweat glands, nerves, and blood vessels. A layer of fatty tissue called the hypodermis lies beneath the dermis and contains blood and lymph vessels (Figure 8.3).
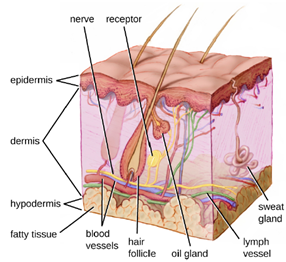
The topmost layer of skin, the epidermis, consists of cells that are packed with keratin. These dead cells remain as a tightly connected, dense layer of protein-filled cell husks on the surface of the skin. The keratin makes the skin’s surface mechanically tough and resistant to degradation by bacterial enzymes. Fatty acids on the skin’s surface create a dry, salty, and acidic environment that inhibits the growth of some microbes and is highly resistant to breakdown by bacterial enzymes. In addition, the dead cells of the epidermis are frequently shed, along with any microbes that may be clinging to them. Shed skin cells are continually replaced with new cells from below, providing a new barrier that will soon be shed in the same way.
Infections can occur when the skin barrier is compromised or broken. A wound can serve as a point of entry for opportunistic pathogens, which can infect the skin tissue surrounding the wound and possibly spread to deeper tissues.
b. Mucous Membranes: The mucous membranes lining the nose, mouth, lungs, and urinary and digestive tracts provide another nonspecific barrier against potential pathogens. Mucous membranes consist of a layer of epithelial cells bound by tight junctions. The epithelial cells secrete a moist, sticky substance called mucus, which covers and protects the more fragile cell layers beneath it and traps debris and particulate matter, including microbes. Mucus secretions also contain antimicrobial peptides.
In many regions of the body, mechanical actions serve to flush mucus (along with trapped or dead microbes) out of the body or away from potential sites of infection. For example, in the respiratory system, inhalation can bring microbes, dust, mold spores, and other small airborne debris into the body. This debris becomes trapped in the mucus lining the respiratory tract, a layer known as the mucociliary blanket. The epithelial cells lining the upper parts of the respiratory tract are called ciliated epithelial cells because they have hair-like appendages known as cilia. Movement of the cilia propels debris-laden mucus out and away from the lungs. The expelled mucus is then swallowed and destroyed in the stomach, or coughed up, or sneezed out (Figure 8.4). This system of removal is often called the mucociliary escalator.
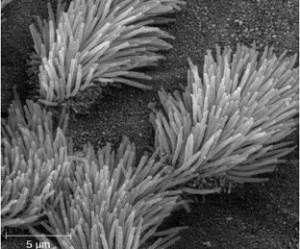
The mucociliary escalator is such an effective barrier to microbes that the lungs, the lowermost (and most sensitive) portion of the respiratory tract, were long considered to be a sterile environment in healthy individuals. Only recently has research suggested that healthy lungs may have a small normal microbiota. Disruption of the mucociliary escalator by the damaging effects of smoking or diseases such as cystic fibrosis can lead to increased colonization of bacteria in the lower respiratory tract and frequent infections, which highlights the importance of this physical barrier to host defenses.
Like the respiratory tract, the digestive tract is a portal of entry through which microbes enter the body, and the mucous membranes lining the digestive tract provide a nonspecific physical barrier against ingested microbes. The intestinal tract is lined with epithelial cells, interspersed with mucus-secreting goblet cells. This mucus mixes with material received from the stomach, trapping foodborne microbes and debris. The mechanical action of peristalsis, a series of muscular contractions in the digestive tract, moves the sloughed mucus and other material through the intestines, rectum, and anus, excreting the material in feces.
c. Endothelia: The epithelial cells lining the urogenital tract, blood vessels, lymphatic vessels, and certain other tissues are known as endothelia. These tightly packed cells provide a particularly effective frontline barrier against invaders. The endothelia of the blood-brain barrier, for example, protect the central nervous system (CNS), which consists of the brain and the spinal cord. The CNS is one of the most sensitive and important areas of the body, as microbial infection of the CNS can quickly lead to serious and often fatal inflammation. The cell junctions in the blood vessels traveling through the CNS are some of the tightest and toughest in the body, preventing any transient microbes in the bloodstream from entering the CNS. This keeps the cerebrospinal fluid that surrounds and bathes the brain and spinal cord sterile under normal conditions.
2. Mechanical Defenses: In addition to physical barriers that keep microbes out, the body has a number of mechanical defenses that physically remove pathogens from the body, preventing them from taking up residence. We have already discussed several examples of mechanical defenses, including the shedding of skin cells, the expulsion of mucus via the mucociliary escalator, and the excretion of feces through intestinal peristalsis. Other important examples of mechanical defenses include the flushing action of urine and tears, which both serve to carry microbes away from the body. The flushing action of urine is largely responsible for the normally sterile environment of the urinary tract, which includes the kidneys, ureters, and urinary bladder. Urine passing out of the body washes out transient microorganisms, preventing them from taking up residence. The eyes also have physical barriers and mechanical mechanisms for preventing infections. The eyelashes and eyelids prevent dust and airborne microorganisms from reaching the surface of the eye. Any microbes or debris that make it past these physical barriers may be flushed out by the mechanical action of blinking, which bathes the eye in tears, washing debris away (Figure 8.5).
3. Microbiome: In various regions of the body, resident microbiota serves as an important first-line defense against invading pathogens. Through their occupation of cellular binding sites and competition for available nutrients, the resident microbiota prevent the critical early steps of pathogen attachment and proliferation required for the establishment of an infection. For example, in the vagina, members of the resident microbiota compete with opportunistic pathogens like the yeast Candida. This competition prevents infections by limiting the availability of nutrients, thus inhibiting the growth of Candida, keeping its population in check. Similar competitions occur between the microbiota and potential pathogens on the skin, in the upper respiratory tract, and in the gastrointestinal tract. As will be discussed later in this chapter, the resident microbiota also contributes to the chemical defenses of the innate nonspecific host defenses.
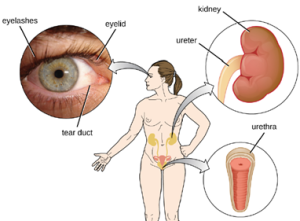
The importance of the normal microbiota in host defenses is highlighted by the increased susceptibility to infectious diseases when the microbiota is disrupted or eliminated. Treatment with antibiotics can significantly deplete the normal microbiota of the gastrointestinal tract, providing an advantage for pathogenic bacteria to colonize and cause diarrheal infection. In the case of diarrhea caused by Clostridioides difficile, the infection can be severe and potentially lethal. One strategy for treating C. difficile infections is fecal transplantation, which involves the transfer of fecal material from a donor (screened for potential pathogens) into the intestines of the recipient patient as a method of restoring the normal microbiota and combating C. difficile infections.
Table 8.2 provides a summary of the physical defenses.
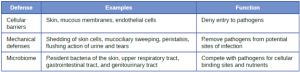
Chemical Defenses: In addition to physical defenses, the innate nonspecific immune system uses a number of chemical mediators that inhibit microbial invaders. The term “chemical mediators” encompasses a wide array of substances found in various body fluids and tissues throughout the body. Chemical mediators may work alone or in conjunction with each other to inhibit microbial colonization and infection.
Some chemical mediators are endogenously produced, meaning they are produced by human body cells; others are produced exogenously, meaning that they are produced by certain microbes that are part of the microbiome. Some mediators are produced continually, bathing the area in the antimicrobial substance; others are produced or activated primarily in response to some stimulus, such as the presence of microbes.
1. Chemical and Enzymatic Mediators Found in Body Fluids: Fluids produced by the skin include examples of both endogenous and exogenous mediators. Sebaceous glands in the dermis secrete an oil called sebum that is released onto the skin surface through hair follicles. This sebum is an endogenous mediator, providing an additional layer of defense by helping seal off the pore of the hair follicle, preventing bacteria on the skin’s surface from invading sweat glands and surrounding tissue (Figure 8.6). Certain members of the microbiome, such as the bacterium Cutibacterium acnes and the fungus Malassezia, among others, can use lipase enzymes to degrade sebum, using it as a food source. This produces oleic acid, which creates a mildly acidic environment on the surface of the skin that is inhospitable to many pathogenic microbes. Oleic acid is an example of an exogenously produced mediator because it is produced by resident microbes and not directly by body cells.
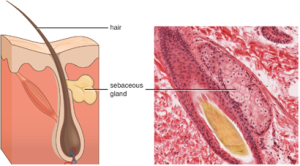
Environmental factors that affect the microbiota of the skin can have a direct impact on the production of chemical mediators. Low humidity or decreased sebum production, for example, could make the skin less habitable for microbes that produce oleic acid, thus making the skin more susceptible to pathogens normally inhibited by the skin’s low pH. Many skin moisturizers are formulated to counter such effects by restoring moisture and essential oils to the skin.
The digestive tract also produces a large number of chemical mediators that inhibit or kill microbes. In the oral cavity, saliva contains mediators such as lactoperoxidase enzymes, and mucus secreted by the esophagus contains the antibacterial enzyme lysozyme. In the stomach, highly acidic gastric fluid kills most microbes. In the lower digestive tract, the intestines have pancreatic and intestinal enzymes, antibacterial peptides (cryptins), bile produced from the liver, and specialized Paneth cells that produce lysozyme. Together, these mediators are able to eliminate most pathogens that manage to survive the acidic environment of the stomach.
In the urinary tract, urine flushes microbes out of the body during urination. Furthermore, the slight acidity of urine (the average pH is about 6) inhibits the growth of many microbes and potential pathogens in the urinary tract.
The female reproductive system employs lactate, an exogenously produced chemical mediator, to inhibit microbial growth. The cells and tissue layers composing the vagina produce glycogen, a branched and more complex polymer of glucose. Lactobacilli in the area ferment glycogen to produce lactate, lowering the pH in the vagina and inhibiting transient microbiota, opportunistic pathogens like Candida (a yeast associated with vaginal infections), and other pathogens responsible for sexually transmitted diseases.
In the eyes, tears contain the chemical mediators lysozyme and lactoferrin, both of which are capable of eliminating microbes that have found their way to the surface of the eyes. Lysozyme cleaves the bond between NAG and NAM in peptidoglycan, a component of the cell wall in bacteria. It is more effective against gram-positive bacteria, which lack the protective outer membrane associated with gram-negative bacteria. Lactoferrin inhibits microbial growth by chemically binding and sequestering iron. This effectually starves many microbes that require iron for growth.
In the ears, cerumen (earwax) exhibits antimicrobial properties due to the presence of fatty acids, which lower the pH to between 3 and 5.
The respiratory tract uses various chemical mediators in the nasal passages, trachea, and lungs. The mucus produced in the nasal passages contains a mix of antimicrobial molecules similar to those found in tears and saliva (e.g., lysozyme, lactoferrin, lactoperoxidase). Secretions in the trachea and lungs also contain lysozyme and lactoferrin, as well as a diverse group of additional chemical mediators, such as the lipoprotein complex called surfactant, which has antibacterial properties
2. Antimicrobial Peptides: The antimicrobial peptides (AMPs) are a special class of nonspecific cell-derived mediators with broad-spectrum antimicrobial properties. Some AMPs are produced routinely by the body, whereas others are primarily produced (or produced in greater quantities) in response to the presence of an invading pathogen. Research has begun exploring how AMPs can be used in the diagnosis and treatment of disease.
AMPs may induce cell damage in microorganisms in a variety of ways, including by inflicting damage to membranes, destroying DNA and RNA, or interfering with cell-wall synthesis. Depending on the specific antimicrobial mechanism, a particular AMP may inhibit only certain groups of microbes (e.g., gram-positive or gram-negative bacteria) or it may be more broadly effective against bacteria, fungi, protozoa, and viruses. Many AMPs are found on the skin, but they can also be found in other regions of the body.
A family of AMPs called defensins can be produced by epithelial cells throughout the body as well as by cellular defenses such as macrophages and neutrophils. Defensins may be secreted or act inside host cells; they combat microorganisms by damaging their plasma membranes. AMPs called bacteriocins are produced exogenously by certain members of the resident microbiota within the gastrointestinal tract. The genes coding for these types of AMPs are often carried on plasmids and can be passed between different species within the resident microbiota through lateral or horizontal gene transfer.
There are numerous other AMPs throughout the body. The characteristics of a few of the more significant AMPs are summarized in Table 8.3.
Table 8.3: Characteristics of Selected Antimicrobial Peptides (AMPs)
AMP | Secreted by | Body site | Pathogens inhibited | Mode of action |
Bacteriocins | Resident microbiota | Gastrointestinal tract | Bacteria | Disrupt membrane |
Cathelicidin | Epithelial cells, macrophages, and other cell types | Skin | Bacteria and fungi | Disrupts membrane |
Defensins | Epithelial cells, macrophages, neutrophils | Throughout the body | Fungi, bacteria, and many viruses | Disrupt membrane |
Dermicidin | Sweat glands | Skin | Bacteria and fungi | Disrupts membrane integrity and ion channels |
Histatins | Salivary glands | Oral cavity | Fungi | Disrupt intracellular function |
2. Plasma Protein Mediators: Many nonspecific innate immune factors are found in plasma, the fluid portion of blood. Plasma contains electrolytes, sugars, lipids, and proteins, each of which helps to maintain homeostasis (i.e., stable internal body functioning), and contains the proteins involved in the clotting of blood. Additional proteins found in blood plasma, such as acute-phase proteins, complement proteins, and cytokines, are involved in the nonspecific innate immune response.
a. Acute-Phase Proteins: The acute-phase proteins are another class of antimicrobial mediators. Acute-phase proteins are primarily produced in the liver and secreted into the blood in response to inflammatory molecules from the immune system. Examples of acute-phase proteins include C-reactive protein, serum amyloid A, ferritin, transferrin, fibrinogen, and mannose-binding lectin. Each of these proteins has a different chemical structure and inhibits or destroys microbes in some way (Table 8.4).
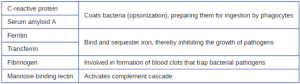
b. The Complement System: The complement system is a group of plasma protein mediators that can act as an innate nonspecific defense while also serving to connect innate and adaptive immunity. The complement system is composed of more than 30 proteins (including C1 through C9) that normally circulate as precursor proteins in blood. These precursor proteins become activated when stimulated or triggered by a variety of factors, including the presence of microorganisms. Complement proteins are considered part of innate nonspecific immunity because they are always present in the blood and tissue fluids, allowing them to be activated quickly. Also, when activated through the alternative pathway (described later in this section), complement proteins target pathogens in a nonspecific manner.
The process by which circulating complement precursors become functional is called complement activation. This process is a cascade that can be triggered by one of three different mechanisms, known as the alternative, classical, and lectin pathways.
The alternative pathway is initiated by the spontaneous activation of the complement protein C3. The hydrolysis of C3 produces two products, C3a and C3b. When no invader microbes are present, C3b is very quickly degraded in a hydrolysis reaction using the water in the blood. However, if invading microbes are present, C3b attaches to the surface of these microbes. Once attached, C3b will recruit other complement proteins in a cascade (Figure 8.7).
The classical pathway provides a more efficient mechanism of activating the complement cascade, but it depends upon the production of antibodies by the specific adaptive immune defenses. To initiate the classical pathway, a specific antibody must first bind to the pathogen to form an antibody-antigen complex. This activates the first protein in the complement cascade, the C1 complex. The C1 complex is a multipart protein complex, and each component participates in the full activation of the overall complex. Following recruitment and activation of the C1 complex, the remaining classical pathway complement proteins are recruited and activated in a cascading sequence (Figure 8.7).
The lectin activation pathway is similar to the classical pathway, but it is triggered by the binding of mannose-binding lectin, an acute-phase protein, to carbohydrates on the microbial surface. Like other acute-phase proteins, lectins are produced by liver cells and are commonly up-regulated in response to inflammatory signals received by the body during an infection (Figure 8.7).
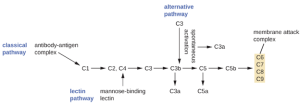
Although each complement activation pathway is initiated in a different way, they all provide the same protective outcomes: opsonization, inflammation, chemotaxis, and cytolysis. The term opsonization refers to the coating of a pathogen by a chemical substance (called an opsonin) that allows phagocytic cells to recognize, engulf, and destroy it more easily. Opsonins from the complement cascade include C1q, C3b, and C4b. Additional important opsonins include mannose-binding proteins and antibodies. The complement fragments C3a and C5a are well-characterized anaphylatoxins with potent proinflammatory functions. Anaphylatoxins activate mast cells, causing degranulation and the release of inflammatory chemical signals, including mediators, such as histamine, that cause vasodilation and increased vascular permeability. C5a is also one of the most potent chemoattractants for neutrophils and other white blood cells, cellular defenses that will be discussed shortly.
The complement proteins C6, C7, C8, and C9 assemble into a membrane attack complex (MAC), which allows C9 to polymerize into pores in the membranes of gram-negative bacteria. These pores allow water, ions, and other molecules to move freely in and out of the targeted cells, eventually leading to cell lysis and death of the pathogen. However, the MAC is only effective against gram-negative bacteria; it cannot penetrate the thick layer of peptidoglycan associated with cell walls of gram-positive bacteria. Since the MAC does not pose a lethal threat to gram-positive bacterial pathogens, complement-mediated opsonization is more important for their clearance.
Certain bacteria also have ways of evading the complement system. Having a capsule can prevent complement activation. Certain capsules contain a monosaccharide called sialic acid which inhibits opsonization and MAC formation. Some gram-negative bacteria have modified LPS which prevents MAC formation. Others lack a capsule, but instead attach sialic acid to their outer membranes. Certain other bacteria produce enzymes which are able to degrade complement proteins, disrupting parts of the pathway.
c. Cytokines: Cytokines are soluble proteins that act as communication signals between cells. In a nonspecific innate immune response, various cytokines may be released to stimulate production of chemical mediators or other cell functions, such as cell proliferation, cell differentiation, inhibition of cell division, apoptosis, and chemotaxis. When a cytokine binds to its target receptor, the effect can vary widely depending on the type of cytokine and the type of cell or receptor to which it has bound. The function of a particular cytokine can be described as autocrine, paracrine, or endocrine (Figure 8.8). In autocrine function, the same cell that releases the cytokine is the recipient of the signal; in other words, autocrine function is a form of self-stimulation by a cell. In contrast, paracrine function involves the release of cytokines from one cell to other nearby cells, stimulating some response from the recipient cells. Last, endocrine function occurs when cells release cytokines into the bloodstream to be carried to target cells much farther away.
Three important classes of cytokines are the interleukins, chemokines, and interferons. The interleukins were originally thought to be produced only by leukocytes (white blood cells) and to only stimulate leukocytes, thus the reasons for their name. Although interleukins are involved in modulating almost every function of the immune system, their role in the body is not restricted to immunity. Interleukins are also produced by and stimulate a variety of cells unrelated to immune defenses.
The chemokines are chemotactic factors that recruit leukocytes to sites of infection, tissue damage, and inflammation. In contrast to more general chemotactic factors, like complement factor C5a, chemokines are very specific in the subsets of leukocytes they recruit.
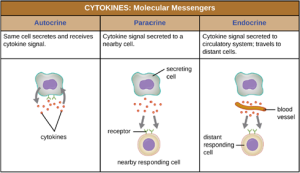
Interferons are a diverse group of immune signaling molecules and are especially important in our defense against viruses. Type I interferons (interferon-α and interferon-β) are produced and released by cells infected with virus. These interferons stimulate nearby cells to stop production of mRNA, destroy RNA already produced, and reduce protein synthesis. These cellular changes inhibit viral replication and production of mature virus, slowing the spread of the virus. Type I interferons also stimulate various immune cells involved in viral clearance to more aggressively attack virus-infected cells. Type II interferon (interferon-γ) is an important activator of immune cells (Figure 8.9).
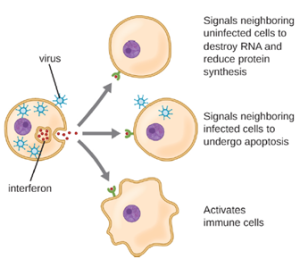
Interferon can be produced in a laboratory and is used as a therapeutic for viral infections and certain cancers. While they can be effective, there are several downsides to using interferons. They only persist in the body for short periods of time, so have a limited effect. They also produce side effects including, nausea, fatigue, headache, vomiting, weight loss and fever. High concentration can also result in damage to the liver, heat, kidneys and red bone marrow. Another issue is, while interferons limit the spread of a viral infection, they have no effect on the viral production of already infected cells.
d. Inflammation-Eliciting Mediators: Many of the chemical mediators discussed in this section contribute in some way to inflammation and fever, which are nonspecific immune responses discussed in more detail in the Inflammation and Fever section below. Cytokines stimulate the production of acute-phase proteins such as C-reactive protein and mannose-binding lectin in the liver. These acute-phase proteins act as opsonins, activating complement cascades through the lectin pathway.
Some cytokines also bind mast cells and basophils, inducing them to release histamine, a proinflammatory compound. Histamine receptors are found on a variety of cells and mediate proinflammatory events, such as bronchoconstriction (tightening of the airways) and smooth muscle contraction.
In addition to histamine, mast cells may release other chemical mediators, such as leukotrienes. Leukotrienes are lipid-based proinflammatory mediators that are produced from the metabolism of arachidonic acid in the cell membrane of leukocytes and tissue cells. Compared with the proinflammatory effects of histamine, those of leukotrienes are more potent and longer lasting. Together, these chemical mediators can induce coughing, vomiting, and diarrhea, which serve to expel pathogens from the body.
Certain cytokines also stimulate the production of prostaglandins, chemical mediators that promote the inflammatory effects of kinins and histamines. Prostaglandins can also help to set the body temperature higher, leading to fever, which promotes the activities of white blood cells and slightly inhibits the growth of pathogenic microbes (see Inflammation and Fever section below).
Another inflammatory mediator, bradykinin, contributes to edema, which occurs when fluids and leukocytes leak out of the bloodstream and into tissues. It binds to receptors on cells in the capillary walls, causing the capillaries to dilate and become more permeable to fluids.
Table 8.5 provides a summary of the chemical defenses discussed in this section.
Cellular Defenses: In the previous section, we discussed some of the chemical mediators found in plasma, the fluid portion of blood. The non-fluid portion of blood consists of various types of formed elements, so called because they are all formed from the same stem cells found in bone marrow. The three major categories of formed elements are: red blood cells (RBCs), also called erythrocytes; platelets, also called thrombocytes; and white blood cells (WBCs), also called leukocytes.
Red blood cells are primarily responsible for carrying oxygen to tissues. Platelets are cellular fragments that participate in blood clot formation and tissue repair. Several different types of WBCs participate in various nonspecific mechanisms of innate and adaptive immunity. In this section, we will focus primarily on the innate mechanisms of various types of WBCs.
Table 8.5: Chemical Defenses of Nonspecific Innate Immunity
All of the formed elements of blood are derived from pluripotent hematopoietic stem cells (HSCs) in the red bone marrow. As the HSCs make copies of themselves in the bone marrow, individual cells receive different cues from the body that control how they develop and mature. As a result, the HSCs differentiate into different types of blood cells that, once mature, circulate in peripheral blood. This process of differentiation, called hematopoiesis, is shown in more detail in Figure 8.10. In terms of sheer numbers, the vast majority of HSCs become erythrocytes. Much smaller numbers become leukocytes and platelets. Leukocytes can be further subdivided into granulocytes, which are characterized by numerous granules visible in the cytoplasm, and agranulocytes, which lack granules.
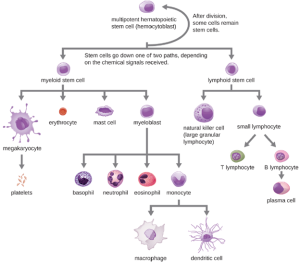
1. Granulocytes: The various types of granulocytes can be distinguished from one another in a blood smear by the appearance of their nuclei and the contents of their granules, which confer different traits, functions, and staining properties. The neutrophils have a nucleus with three to five lobes and small, numerous, lilac-colored granules. Each lobe of the nucleus is connected by a thin strand of material to the other lobes. The eosinophils have fewer lobes in the nucleus (typically 2–3) and larger granules that stain reddish-orange. The basophils have a two-lobed nucleus and large granules that stain dark blue or purple (Figure 8.11).
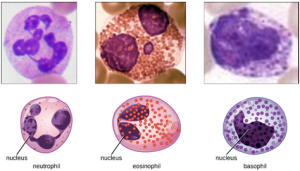
a. Neutrophils: Neutrophils are frequently involved in the elimination and destruction of extracellular bacteria. They are capable of migrating through the walls of blood vessels to areas of bacterial infection and tissue damage, where they seek out and kill infectious bacteria. Neutrophils granules contain a variety of defensins and hydrolytic enzymes that help them destroy bacteria through phagocytosis. In addition, when many neutrophils are brought into an infected area, they can be stimulated to release toxic molecules into the surrounding tissue to better clear infectious agents. This is called degranulation.
Another mechanism used by neutrophils is neutrophil extracellular traps (NETs), which are extruded meshes of chromatin that are closely associated with antimicrobial granule proteins and components. Chromatin is DNA with associated proteins. By creating and releasing a mesh or lattice-like structure of chromatin that is coupled with antimicrobial proteins, the neutrophils can mount a highly concentrated and efficient attack against nearby pathogens. As neutrophils fight an infection, a visible accumulation of leukocytes, cellular debris, and bacteria at the site of infection can be observed. This buildup is what we call pus (also known as purulent or suppurative discharge or drainage). The presence of pus is a sign that the immune defenses have been activated against an infection.
b. Eosinophils: Eosinophils are granulocytes that protect against protozoa and helminths; they also play a role in allergic reactions. The granules of eosinophils, contain histamine, degradative enzymes, and a compound known as major basic protein (MBP). MBP binds to the surface carbohydrates of parasites, and this binding is associated with disruption of the cell membrane and membrane permeability.
c. Basophils: Activated complement fragments C3a and C5a, produced in the activation cascades of complement proteins, act as anaphylatoxins by inducing degranulation of basophils and inflammatory responses. This cell type is important in allergic reactions and other responses that involve inflammation. One of the most abundant components of basophil granules is histamine, which is released along with other chemical factors when the basophil is stimulated. These chemicals can be chemotactic and can help to open the gaps between cells in the blood vessels.
d. Mast Cells: Hematopoiesis also gives rise to mast cells, which appear to be derived from the same common myeloid progenitor cell as neutrophils, eosinophils, and basophils. Functionally, mast cells are very similar to basophils, containing many of the same components in their granules (e.g., histamine) and playing a similar role in allergic responses and other inflammatory reactions. However, unlike basophils, mast cells leave the circulating blood and are most frequently found residing in tissues. They are often associated with blood vessels and nerves or found close to surfaces that interface with the external environment, such as the skin and mucous membranes in various regions of the body
2. Agranulocytes: As their name suggests, agranulocytes lack visible granules in the cytoplasm. Agranulocytes can be categorized as lymphocytes or monocytes. Among the lymphocytes are natural killer cells, which play an important role in nonspecific innate immune defenses. Lymphocytes also include the B cells and T cells, which are discussed later because they are central players in the specific adaptive immune defenses. The monocytes differentiate into macrophages and dendritic cells, which are collectively referred to as the mononuclear phagocyte system.
a. Natural Killer Cells: Most lymphocytes are primarily involved in the specific adaptive immune response, and thus will be discussed in the following chapter. An exception is the natural killer cells (NK cells); these mononuclear lymphocytes use nonspecific mechanisms to recognize and destroy cells that are abnormal in some way. Cancer cells and cells infected with viruses are two examples of cellular abnormalities that are targeted by NK cells. Recognition of such cells involves a complex process of identifying inhibitory and activating molecular markers on the surface of the target cell. Molecular markers that make up the major histocompatibility complex (MHC) are expressed by healthy cells as an indication of “self.” This will be covered in more detail in next chapter. NK cells are able to recognize normal MHC markers on the surface of healthy cells, and these MHC markers serve as an inhibitory signal preventing NK cell activation. However, cancer cells and virus-infected cells actively diminish or eliminate expression of MHC markers on their surface. When these MHC markers are diminished or absent, the NK cell interprets this as an abnormality and a cell in distress. This is one part of the NK cell activation process (Figure 8.12). NK cells are also activated by binding to activating molecular molecules on the target cell. These activating molecular molecules include “altered self” or “nonself” molecules. When a NK cell recognizes a decrease in inhibitory normal MHC molecules and an increase in activating molecules on the surface of a cell, the NK cell will be activated to eliminate the cell in distress.
Once a cell has been recognized as a target, the NK cell can use several different mechanisms to kill its target. For example, it may express cytotoxic membrane proteins and cytokines that stimulate the target cell to undergo apoptosis, or controlled cell suicide. NK cells may also use perforin-mediated cytotoxicity to induce apoptosis in target cells. This mechanism relies on two toxins released from granules in the cytoplasm of the NK cell: perforin, a protein that creates pores in the target cell, and granzymes, proteases that enter through the pores into the target cell’s cytoplasm, where they trigger a cascade of protein activation that leads to apoptosis. The NK cell binds to the abnormal target cell, releases its destructive payload, and detaches from the target cell. While the target cell undergoes apoptosis, the NK cell synthesizes more perforin and proteases to use on its next target.
b. Monocytes: The largest of the white blood cells, monocytes have a nucleus that lacks lobes, and they also lack granules in the cytoplasm (Figure 8.13). Nevertheless, they are effective phagocytes, engulfing pathogens and apoptotic cells to help fight infection.
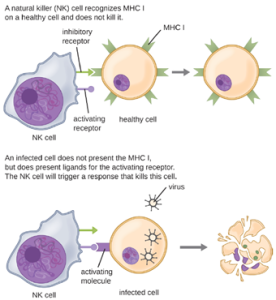
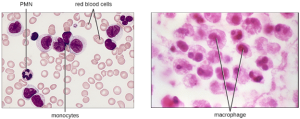
When monocytes leave the bloodstream and enter a specific body tissue, they differentiate into tissue-specific phagocytes called macrophages and dendritic cells. They are particularly important residents of lymphoid tissue, as well as non-lymphoid sites and organs. Macrophages and dendritic cells can reside in body tissues for significant lengths of time. Macrophages in specific body tissues develop characteristics suited to the particular tissue. Not only do they provide immune protection for the tissue in which they reside but they also support normal function of their neighboring tissue cells through the production of cytokines. Dendritic cells are important sentinels residing in the skin and mucous membranes, which are portals of entry for many pathogens. Monocytes, macrophages, and dendritic cells are all highly phagocytic and important promoters of the immune response through their production and release of cytokines. These cells provide an essential bridge between innate and adaptive immune responses, as discussed in the next section as well as the next chapter.
Pathogen Recognition and Phagocytosis: Several of the cell types discussed in the previous section can be described as phagocytes—cells whose main function is to seek, ingest, and kill pathogens. Phagocytes provide a strong, swift, and effective defense against a broad range of microbes, making them a critical component of innate nonspecific immunity. This section will focus on the mechanisms by which phagocytes are able to seek, recognize, and destroy pathogens.
1. Extravasation (Diapedesis) of Leukocytes: Some phagocytes are leukocytes (WBCs) that normally circulate in the bloodstream. To reach pathogens located in infected tissue, leukocytes must pass through the walls of small capillary blood vessels within tissues. This process, called extravasation, or diapedesis, is initiated by complement factor C5a, as well as cytokines released into the immediate vicinity by resident macrophages and tissue cells responding to the presence of the infectious agent (Figure 8.14). Similar to C5a, many of these cytokines are proinflammatory and chemotactic (acting as chemoattractants to recruit phagocytes), and they bind to cells of small capillary blood vessels, initiating a response in the endothelial cells lining the inside of the blood vessel walls. This response involves the upregulation and expression of various cellular adhesion molecules and receptors. Leukocytes passing through will stick slightly to the adhesion molecules, slowing down and rolling along the blood vessel walls near the infected area. When they reach a cellular junction, they will bind to even more of these adhesion molecules, flattening out and squeezing through the cellular junction in a process known as transendothelial migration. This mechanism of “rolling adhesion” allows leukocytes to exit the bloodstream and enter the infected areas, where they can begin phagocytizing the invading pathogens.
Note that extravasation does not occur in arteries or veins. These blood vessels are surrounded by thicker, multilayer protective walls, in contrast to the thin single-cell-layer walls of capillaries. Furthermore, the blood flow in arteries is too turbulent to allow for rolling adhesion. Also, some leukocytes tend to respond to an infection more quickly than others. The first to arrive typically are neutrophils, often within hours of a bacterial infection. By contract, monocytes may take several days to leave the bloodstream and differentiate into macrophages.
2. Pathogen Recognition: As described in the previous section, opsonization of pathogens by antibody; complement factors C1q, C3b, and C4b; and lectins can assist phagocytic cells in recognition of pathogens and attachment to initiate phagocytosis. However, not all pathogen recognition is opsonin dependent. Phagocytes can also recognize molecular structures that are common to many groups of pathogenic microbes. Such structures are called pathogen-associated molecular patterns (PAMPs). Common PAMPs include the following:
· peptidoglycan, found in bacterial cell walls;
· flagellin, a protein found in bacterial flagella;
· lipopolysaccharide (LPS) from the outer membrane of gram-negative bacteria;
· lipopeptides, molecules expressed by most bacteria; and
· nucleic acids such as viral DNA or RNA.
Like numerous other PAMPs, these substances are integral to the structure of broad classes of microbes.
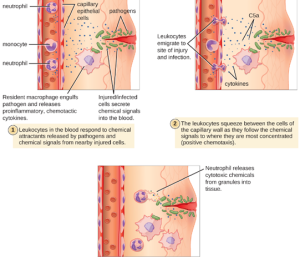
The structures that allow phagocytic cells to detect PAMPs are called pattern recognition receptors (PRRs). One group of PRRs is the toll-like receptors (TLRs), which bind to various PAMPs and communicate with the nucleus of the phagocyte to elicit a response. Many TLRs (and other PRRs) are located on the surface of a phagocyte, but some can also be found embedded in the membranes of interior compartments and organelles (Figure 8.25). These interior PRRs can be useful for the binding and recognition of intracellular pathogens that may have gained access to the inside of the cell before phagocytosis could take place. Viral nucleic acids, for example, might encounter an interior PRR, triggering production of the antiviral cytokine interferon.
In addition to providing the first step of pathogen recognition, the interaction between PAMPs and PRRs on macrophages provides an intracellular signal that activates the phagocyte, causing it to transition from a dormant state of readiness and slow proliferation to a state of hyperactivity, proliferation, production/secretion of cytokines, and enhanced intracellular killing. PRRs on macrophages also respond to chemical distress signals from damaged or stressed cells. This allows macrophages to extend their responses beyond protection from infectious diseases to a broader role in the inflammatory response initiated from injuries or other diseases.
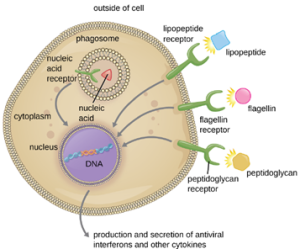
3. Pathogen Degradation: Once pathogen recognition and attachment occurs, the pathogen is engulfed in a vesicle and brought into the internal compartment of the phagocyte in a process called phagocytosis (Figure 8.16). PRRs can aid in phagocytosis by first binding to the pathogen’s surface, but phagocytes are also capable of engulfing nearby items even if they are not bound to specific receptors. To engulf the pathogen, the phagocyte forms a pseudopod that wraps around the pathogen and then pinches it off into a membrane vesicle called a phagosome. Acidification of the phagosome (pH decreases to the range of 4–5) provides an important early antibacterial mechanism. The phagosome containing the pathogen fuses with one or more lysosomes, forming a phagolysosome. Formation of the phagolysosome enhances the acidification, which is essential for activation of pH-dependent digestive lysosomal enzymes and production of hydrogen peroxide and toxic reactive oxygen species. Lysosomal enzymes such as lysozyme, phospholipase, and proteases digest the pathogen. Other enzymes are involved a respiratory burst. During the respiratory burst, phagocytes will increase their uptake and consumption of oxygen, but not for energy production. The increased oxygen consumption is focused on the production of superoxide anion, hydrogen peroxide, hydroxyl radicals, and other reactive oxygen species that are antibacterial.
In addition to the reactive oxygen species produced by the respiratory burst, reactive nitrogen compounds with cytotoxic (cell-killing) potential can also form. For example, nitric oxide can react with superoxide to form peroxynitrite, a highly reactive nitrogen compound with degrading capabilities similar to those of the reactive oxygen species. Some phagocytes even contain an internal storehouse of microbicidal defensin proteins (e.g., neutrophil granules). These destructive forces can be released into the area around the cell to degrade microbes externally. Neutrophils, especially, can be quite efficient at this secondary antimicrobial mechanism.
Once degradation is complete, leftover waste products are excreted from the cell in an exocytic vesicle. However, it is important to note that not all remains of the pathogen are excreted as waste. Macrophages and dendritic cells are also antigen-presenting cells involved in the specific adaptive immune response. These cells further process the remains of the degraded pathogen and present key antigens (specific pathogen proteins) on their cellular surface. This is an important step for stimulation of some adaptive immune responses.
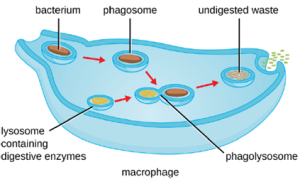
As has been mentioned previously, certain virulence factors allow for pathogens to evade being destroyed via phagocytosis. Some bacteria such as Streptococcus pneumoniae and Haemophilus influenzae have capsules, while others, such as Streptococcus pyogenes, contain M protein in their cell walls. These factors inhibit adherence of the phagocyte to the pathogen, thereby reducing the likelihood of it being phagocytized. Some bacteria are ingested but not digested. Staphylococcus aureus produces molecules that cause the phagocyte to release its own digestive enzymes into its cytoplasm, causing it to die. Still other pathogens actually survive and replicate inside the phagocytes after being digested. This is facilitated either by the pathogen escaping the phagosome and entering the cytoplasm, such is the case with Shigella, or by preventing the fusion of lysosomes to the phagosome and replicating inside phagosome, as with Plasmodium and Mycobacterium tuberculosis. Biofilms also help to prevent phagocytosis because phagocytes have difficulty in detaching pathogens from the biofilm so they can be ingested.
Inflammation and Fever: The inflammatory response, or inflammation, is triggered by a cascade of chemical mediators and cellular responses that may occur when cells are damaged and stressed or when pathogens successfully breach the physical barriers of the innate immune system. Although inflammation is typically associated with negative consequences of injury or disease, it is a necessary process insofar as it allows for recruitment of the cellular defenses needed to eliminate pathogens, remove damaged and dead cells, and initiate repair mechanisms. Excessive inflammation, however, can result in local tissue damage and, in severe cases, may even become deadly.
1. Acute Inflammation: An early, if not immediate, response to tissue injury is acute inflammation. Immediately following an injury, vasoconstriction of blood vessels will occur to minimize blood loss. The amount of vasoconstriction is related to the amount of vascular injury, but it is usually brief. Vasoconstriction is followed by vasodilation and increased vascular permeability, as a direct result of the release of histamine from resident mast cells. Increased blood flow and vascular permeability can dilute toxins and bacterial products at the site of injury or infection. They also contribute to the five observable signs associated with the inflammatory response: erythema (redness), edema (swelling), heat, pain, and altered function. Vasodilation and increased vascular permeability are also associated with an influx of phagocytes at the site of injury and/or infection. This can enhance the inflammatory response because phagocytes may release proinflammatory chemicals when they are activated by cellular distress signals released from damaged cells, by PAMPs, or by opsonins on the surface of pathogens. Activation of the complement system can further enhance the inflammatory response through the production of the anaphylatoxin C5a. Figure 8.17 illustrates a typical case of acute inflammation at the site of a skin wound.
During the period of inflammation, the release of bradykinin causes capillaries to remain dilated, flooding tissues with fluids and leading to edema. Increasing numbers of neutrophils are recruited to the area to fight pathogens. As the fight rages on, pus forms from the accumulation of neutrophils, dead cells, tissue fluids, and lymph. Typically, after a few days, macrophages will help to clear out this pus. Eventually, tissue repair can begin in the wounded area.
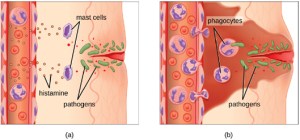
2. Chronic Inflammation: When acute inflammation is unable to clear an infectious pathogen, chronic inflammation may occur. This often results in an ongoing (and sometimes futile) lower-level battle between the host organism and the pathogen. The wounded area may heal at a superficial level, but pathogens may still be present in deeper tissues, stimulating ongoing inflammation. Additionally, chronic inflammation may be involved in the progression of degenerative neurological diseases such as Alzheimer’s and Parkinson’s, heart disease, and metastatic cancer.
Chronic inflammation may lead to the formation of granulomas, pockets of infected tissue walled off and surrounded by WBCs. Macrophages and other phagocytes wage an unsuccessful battle to eliminate the pathogens and dead cellular materials within a granuloma. One example of a disease that produces chronic inflammation is tuberculosis, which results in the formation of granulomas in lung tissues. A tubercular granuloma is called a tubercle (Figure 8.18).
Chronic inflammation is not just associated with bacterial infections. Chronic inflammation can be an important cause of tissue damage from viral infections. The extensive scarring observed with hepatitis C infections and liver cirrhosis is the result of chronic inflammation.
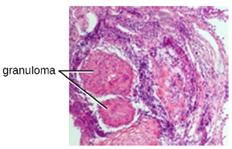
- Fever: A fever is an inflammatory response that extends beyond the site of infection and affects the entire body, resulting in an overall increase in body temperature. Body temperature is normally regulated and maintained by the hypothalamus, an anatomical section of the brain that functions to maintain homeostasis in the body. However, certain bacterial or viral infections can result in the production of pyrogens, chemicals that effectively alter the “thermostat setting” of the hypothalamus to elevate body temperature and cause fever. Pyrogens may be exogenous or endogenous. For example, the endotoxin lipopolysaccharide (LPS), produced by gram-negative bacteria, is an exogenous pyrogen that may induce the leukocytes to release endogenous pyrogens such as interleukin-1 (IL-1), IL-6, interferon-γ (IFN-γ), and tumor necrosis factor (TNF). In a cascading effect, these molecules can then lead to the release of prostaglandin E2 (PGE2) from other cells, resetting the hypothalamus to initiate fever (Figure 8.19).
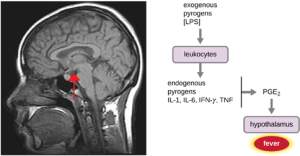
Like other forms of inflammation, a fever enhances the innate immune defenses by stimulating leukocytes to kill pathogens. The rise in body temperature also may inhibit the growth of many pathogens since human pathogens are mesophiles with optimum growth occurring around 35 °C (95 °F). In addition, some studies suggest that fever may also stimulate release of iron-sequestering compounds from the liver, thereby starving out microbes that rely on iron for growth.
During fever, the skin may appear pale due to vasoconstriction of the blood vessels in the skin, which is mediated by the hypothalamus to divert blood flow away from extremities, minimizing the loss of heat and raising the core temperature. The hypothalamus will also increase metabolism and stimulate shivering of muscles, which are other effective mechanisms of generating heat and raising the core temperature.
The crisis phase occurs when the fever breaks. The hypothalamus stimulates vasodilation, resulting in a return of blood flow to the skin and a subsequent release of heat from the body. The hypothalamus also stimulates sweating, which cools the skin as the sweat evaporates.
Although a low-level fever may help an individual overcome an illness, in some instances, this immune response can be too strong, causing tissue and organ damage and, in severe cases, even death. Other complications of fever include: tachycardia (rapid heart rate), metabolic acidosis, dehydration, seizures, delirium and coma. The inflammatory response to bacterial superantigens is one scenario in which a life-threatening fever may develop. Superantigens are bacterial or viral proteins that can cause an excessive activation of T cells from the specific adaptive immune defense, as well as an excessive release of cytokines that overstimulates the inflammatory response. For example, Staphylococcus aureus and Streptococcus pyogenes are capable of producing superantigens that cause toxic shock syndrome and scarlet fever, respectively. Both of these conditions can be associated with very high, life-threatening fevers in excess of 42 °C (108 °F).