Unit 2.2 The Structure of Prokaryotic and Eukaryotic Cells
Outline
Unique Characteristics of Prokaryotic Cells
- The Nucleoid
- Plasmids
- Ribosomes
- Inclusions
- Endospores
- Plasma Membrane
- Cell Wall
- Glycocalyces
- Filamentous Appendages
- Cell Division
- Genetic Variation
Unique Characteristics of Eukaryotic Cells
- Nucleus
- Ribosomes
- Endomembrane System and Other Organelles
- Cytoskeleton
- Plasma Membrane
- Cell Wall
- Extracellular Matrix
- Flagella and Cilia
- Cell Division
Learning Objectives
After reading the following, you should be able to:
- Define prokaryotes and eukaryotes and describe the key features that distinguish them. This includes:
- Areas where DNA is held, and the forms in which DNA exists (including plasmids)
- Cell division
- Genetic variation
- Protein synthesis
- Plasma membranes
- Cell wall
- Cell movement
- Structures only prokaryotes have (inclusions, endospores, glycocalyx, fimbriae, and pili)
- Structures only eukaryotes have (Cytoskeleton, membranous organelles, cilia and extracellular matrix)
All life on Earth evolved from a common ancestor. Biologists map how organisms are related by constructing phylogenetic trees. In other words, a “tree of life” can be constructed to illustrate when different organisms evolved and to show the relationships among different organisms, as shown in Figure 2.8. Notice that from a single point, the three domains of Archaea, Bacteria, and Eukarya diverge and then branch repeatedly. The small branch that plants and animals (including humans) occupy in this diagram shows how recently these groups had their origin compared with other groups.
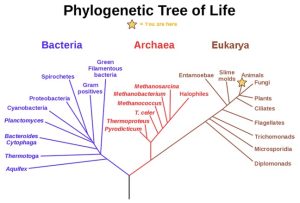
Unique Characteristics of Prokaryotic Cells
All living things fit into one of these 3 categories and share some fundamental cellular similarities. These include cytoplasm (a gel-like substance composed of water and dissolved chemicals needed for growth), which is contained within a plasma membrane (also called a cell membrane or cytoplasmic membrane); one or more chromosomes, which contain the genetic blueprints of the cell; and ribosomes, organelles used for the production of proteins.
Beyond these basic components, cells can vary greatly between organisms, and even within the same multicellular organism. The two largest categories of cells—prokaryotic cells and eukaryotic cells—are defined by major differences in several cell structures. Prokaryotic cells lack a nucleus surrounded by a complex nuclear membrane and generally have a single, circular chromosome located in a region of the cytoplasm called the nucleoid. Eukaryotic cells have a nucleus surrounded by a complex nuclear membrane that contains multiple, linear chromosomes.
All plant cells and animal cells are eukaryotic. Some microorganisms are composed of prokaryotic cells, whereas others are composed of eukaryotic cells. Prokaryotic microorganisms are classified within the domains Archaea and Bacteria, whereas eukaryotic organisms are classified within the domain Eukarya.
The structures inside a cell are analogous to the organs inside a human body, with unique structures suited to specific functions. Some of the structures found in prokaryotic cells are similar to those found in some eukaryotic cells; others are unique to prokaryotes. Although there are some exceptions, eukaryotic cells tend to be larger than prokaryotic cells. The comparatively larger size of eukaryotic cells dictates the need to compartmentalize various chemical processes within different areas of the cell, using complex membrane-bound organelles. In contrast, prokaryotic cells generally lack membrane-bound organelles; however, they often contain inclusions that compartmentalize their cytoplasm. Figure 2.9 illustrates structures typically associated with prokaryotic cells. These structures are described in more detail in the next section.
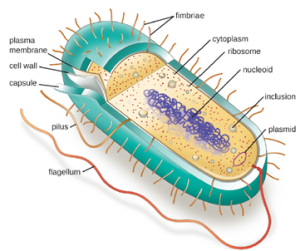
The Nucleoid: All cellular life has a DNA genome organized into one or more chromosomes. Prokaryotic chromosomes are typically circular, haploid (unpaired), and not bound by a complex nuclear membrane. Prokaryotic DNA and DNA-associated proteins are concentrated within the nucleoid region of the cell (Figure 2.10). In general, prokaryotic DNA interacts with nucleoid-associated proteins (NAPs) that assist in the organization and packaging of the chromosome. In bacteria, NAPs function similar to histones, which are the DNA-organizing proteins found in eukaryotic cells.
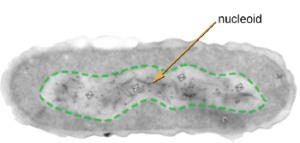
Plasmids: Prokaryotic cells may also contain extrachromosomal DNA, or DNA that is not part of the chromosome. This extrachromosomal DNA is found in plasmids, which are small, circular, double-stranded DNA molecules. Cells that have plasmids often have hundreds of them within a single cell. Plasmids are more commonly found in bacteria; however, plasmids have been found in archaea and eukaryotic organisms. Plasmids often carry genes that confer advantageous traits such as antibiotic resistance; thus, they are important to the survival of the organism.
Ribosomes: All cellular life synthesizes proteins, and organisms in all three domains of life possess ribosomes, structures responsible protein synthesis. However, ribosomes in each of the three domains are structurally different. Ribosomes, themselves, are constructed from proteins, along with ribosomal RNA (rRNA). Prokaryotic ribosomes are found in the cytoplasm. They are called 70S ribosomes because they have a size of 70S, whereas eukaryotic cytoplasmic ribosomes have a size of 80S. (The S stands for Svedberg unit, a measure of sedimentation in an ultracentrifuge, which is based on size, shape, and surface qualities of the structure being analyzed). Although they are the same size, bacterial and archaeal ribosomes have different proteins and rRNA molecules, and the archaeal versions are more similar to their eukaryotic counterparts than to those found in bacteria.
Inclusions: As single-celled organisms living in unstable environments, some prokaryotic cells have the ability to store excess nutrients within cytoplasmic structures called inclusions. Storing nutrients in a polymerized form is advantageous because it reduces the buildup of osmotic pressure that occurs as a cell accumulates solutes. Various types of inclusions store glycogen and starches, which contain carbon that cells can access for energy. Metachromatic granules because of their staining characteristics, are inclusions that store polymerized inorganic phosphate that can be used in metabolism and assist in the formation of biofilms. Sulfur granules, another type of inclusion, these granules store elemental sulfur, which the bacteria use for metabolism.
Some prokaryotic cells have other types of inclusions that serve purposes other than nutrient storage. For example, some prokaryotic cells produce gas vacuoles, accumulations of small, protein-lined vesicles of gas. These gas vacuoles allow the prokaryotic cells that synthesize them to alter their buoyancy so that they can adjust their location in the water column. Magnetotactic bacteria, contain magnetosomes, which are inclusions of magnetic iron oxide or iron sulfide surrounded by a lipid layer. These allow cells to align along a magnetic field, aiding their movement.
Endospores: Bacterial cells are generally observed as vegetative cells, but some genera of bacteria have the ability to form endospores, structures that essentially protect the bacterial genome in a dormant state when environmental conditions are unfavorable. Endospores (not to be confused with the reproductive spores formed by fungi) allow some bacterial cells to survive long periods without food or water, as well as exposure to chemicals, extreme temperatures, and even radiation. Table 3.1 compares the characteristics of vegetative cells and endospores.
Vegetative Cells | Endospores |
---|---|
Sensitive to extreme temperatures and radiation | Resistant to extreme temperatures and radiation |
Gram positive | Do not absorb Gram stain, only special endospore stains |
Normal water content and enzymatic activity | Dehydrated; no metabolic activity |
Capable of active growth and metabolism | Dormant; no growth or metabolic activity |
The process by which vegetative cells transform into endospores is called sporulation, and it generally begins when nutrients become depleted or environmental conditions become otherwise unfavorable. Endospores of certain species have been shown to persist in a dormant state for extended periods of time, up to thousands of years. However, when living conditions improve, endospores undergo germination, reentering a vegetative state. After germination, the cell becomes metabolically active again and is able to carry out all of its normal functions, including growth and cell division (Figure 2.11).
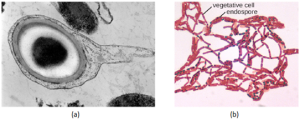
Not all bacteria have the ability to form endospores; however, there are a number of clinically significant endospore forming gram-positive bacteria of the genera Bacillus and Clostridium. These include B. anthracis, the causative agent of anthrax, which produces endospores capable of survive for many decades; C. tetani (causes tetanus); C. difficile (causes pseudomembranous colitis); C. perfringens (causes gas gangrene); and C. botulinum (causes botulism). Pathogens such as these are particularly difficult to combat because their endospores are so hard to kill.
Plasma Membrane: Structures that enclose the cytoplasm and internal structures of the cell are known collectively as the cell envelope. In prokaryotic cells, the structures of the cell envelope vary depending on the type of cell and organism. Most (but not all) prokaryotic cells have a cell wall, but the makeup of this cell wall varies. All cells (prokaryotic and eukaryotic) have a plasma membrane (also called cytoplasmic membrane or cell membrane) that exhibits selective permeability, allowing some molecules to enter or leave the cell while restricting the passage of others.
The structure of the plasma membrane is often described in terms of the fluid mosaic model, which refers to the ability of membrane components to move fluidly within the plane of the membrane, as well as the mosaic-like composition of the components, which include a diverse array of lipid and protein components. The plasma membrane structure of most bacterial and eukaryotic cell types is a bilayer composed mainly of phospholipids formed with ester linkages and proteins. These phospholipids and proteins have the ability to move laterally within the plane of the membranes as well as between the two phospholipid layers. Prokaryotic cell membranes also lack sterols, which are found in Eukaryotic plasma membrane to help to regulate membrane fluidity.
Cell Wall: The primary function of the cell wall is to protect the cell from harsh conditions in the outside environment. When present, there are notable similarities and differences among the cell walls of archaea, bacteria, and eukaryotes.
The major component of bacterial cell walls is called peptidoglycan; it is only found in bacteria.
Structurally, peptidoglycan resembles a layer of meshwork or fabric (Figure 2.12). Each layer is composed of long chains of alternating molecules of N-acetylglucosamine (NAG) and N-acetylmuramic acid (NAM). The structure of the long chains has significant two-dimensional tensile strength due to the formation of peptide bridges that connect NAG and NAM within each peptidoglycan layer. Peptidoglycan subunits are made inside of the bacterial cell and then exported and assembled in layers, giving the cell its shape.
Since peptidoglycan is unique to bacteria, many antibiotic drugs are designed to interfere with peptidoglycan synthesis, weakening the cell wall and making bacterial cells more susceptible to the effects of osmotic pressure. In addition, certain cells of the human immune system are able to “recognize” bacterial pathogens by detecting peptidoglycan on the surface of a bacterial cell; these cells then engulf and destroy the bacterial cell, using enzymes such as lysozyme, which breaks down and digests the peptidoglycan in their cell walls.
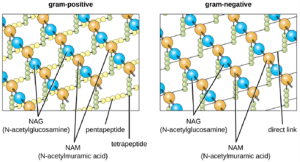
The Gram staining protocol (Discussed in detail later) is used to differentiate two common types of cell wall structures (Figure 2.13). Gram-positive cells have a cell wall consisting of many layers of peptidoglycan totaling 30–100 nm in thickness. These peptidoglycan layers are commonly embedded with teichoic acids (TAs), carbohydrate chains that extend through and beyond the peptidoglycan layer. There are two classes of TAs, wall teichoic acids that are linked to the peptidoglycan layer and lipoteichoic acids, which are bound to the plasma membrane and span the cell wall. TA is thought to stabilize peptidoglycan by increasing its rigidity. Because they are negatively charged, TAs also play a role in helping regulate the movement of ions through the cell wall. TA also plays a role in the ability of pathogenic gram-positive bacteria such as Streptococcus to bind to certain proteins on the surface of host cells, enhancing their ability to cause infection.
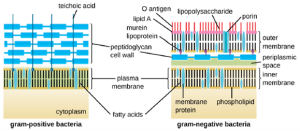
Gram-negative cells have a much thinner layer of peptidoglycan (no more than about 4 nm thick) than Gram positive cells, and the overall structure of their cell envelope is more complex. In gram-negative cells, a gel-like matrix occupies the periplasmic space between the cell wall and the plasma membrane, and there is a second lipid bilayer called the outer membrane, which is external to the peptidoglycan layer (Figure 2.13). This outer membrane is attached to the peptidoglycan by murein lipoprotein. The outer leaflet of the outer membrane contains the molecule lipopolysaccharide (LPS), which functions as an endotoxin in infections involving gram-negative bacteria, contributing to symptoms such as fever, hemorrhaging, and septic shock. Each LPS molecule is composed of Lipid A, a core polysaccharide, and an O side chain that is composed of sugar-like molecules that comprise the external face of the LPS. The composition of the O side chain varies between different species and strains of bacteria. Parts of the O side chain called antigens can be detected using serological or immunological tests to identify specific pathogenic strains like Escherichia coli O157:H7, a deadly strain of bacteria that causes bloody diarrhea and kidney failure.
Archaeal cell wall structure differs from that of bacteria in several significant ways. First, archaeal cell walls do not contain peptidoglycan; instead, they contain a similar polymer called pseudopeptidoglycan in which NAM is replaced with a different subunit. Other archaea may have a layer of glycoproteins or polysaccharides that serves as the cell wall instead of pseudopeptidoglycan. Last, as is the case with some bacterial species, there are a few archaea that appear to lack cell walls entirely.
Glycocalyces: A glycocalyx is a sugar coat, of which there are two important types: capsules and slime layers. A capsule is an organized layer located outside of the cell wall and usually composed of polysaccharides or proteins (Figure 2.14). A slime layer is a less tightly organized layer that is only loosely attached to the cell wall and can be more easily washed off. Slime layers may be composed of polysaccharides, glycoproteins, or glycolipids.
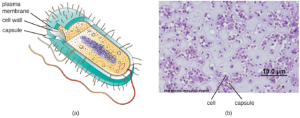
The ability to produce a capsule can contribute to a microbe’s pathogenicity (ability to cause disease) because the capsule can make it more difficult for phagocytic cells (such as white blood cells) to engulf and kill the microorganism. Streptococcus pneumoniae, for example, produces a capsule that is well known to aid in this bacterium’s pathogenicity. Capsules are difficult to stain for microscopy; negative staining techniques are typically used.
Many bacterial cells have protein appendages embedded within their cell envelopes that extend outward, allowing interaction with the environment. These appendages can attach to other surfaces, transfer DNA, or provide movement. Filamentous appendages include fimbriae, pili, and flagella.
Fimbriae and Pili: Fimbriae and pili are structurally similar and, because differentiation between the two is problematic, these terms are often used interchangeably. The term fimbriae commonly refers to short bristle-like proteins projecting from the cell surface by the hundreds. Fimbriae enable a cell to attach to surfaces and to other cells. For pathogenic bacteria, adherence to host cells is important for colonization, infectivity, and virulence. Adherence to surfaces is also important in biofilm formation.
The term pili (singular: pilus) commonly refers to longer, less numerous protein appendages that aid in attachment to surfaces (Figure 2.15). A specific type of pilus, called the F pilus or sex pilus, is important in the transfer of DNA between bacterial cells, which occurs between members of the same generation when two cells physically transfer or exchange parts of their respective genomes.
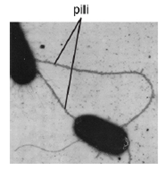
Flagella: Flagella are structures used by cells to move in aqueous environments. Bacterial flagella act like propellers. They are stiff spiral filaments composed of flagellin protein subunits that extend outward from the cell and spin in solution. The basal body is the motor for the flagellum and is embedded in the plasma membrane (Figure 2.16). A hook region connects the basal body to the filament. Gram-positive and gram-negative bacteria have different basal body configurations due to differences in cell wall structure.
Different types of motile bacteria exhibit different arrangements of flagella (Figure 2.17). A bacterium with a singular flagellum, typically located at one end of the cell (polar), is said to have a monotrichous flagellum. An example of a monotrichously flagellated bacterial pathogen is Vibrio cholerae, the gram-negative bacterium that causes cholera. Cells with amphitrichous flagella have a flagellum at each end. An example is Spirillum minor, the cause of spirillary (Asian) rat-bite fever or sodoku. Cells with lophotrichous flagella have a tuft at one end of the cell. The gram-negative bacillus Pseudomonas aeruginosa, an opportunistic pathogen known for causing many infections, including “swimmer’s ear” and burn wound infections, has lophotrichous flagella. Flagella that cover the entire surface of a bacterial cell are called peritrichous flagella. The gram-negative bacterium E. coli shows a peritrichous arrangement of flagella. If a bacterium lacks any flagella it is atrichous.
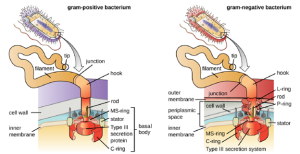
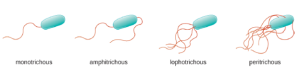
Directional movement depends on the configuration of the flagella. Bacteria can move in response to a variety of environmental signals. Movement in response to an external stimulus is known as taxis. Movement towards a stimulus is known as positive taxis and movement away from a stimulus called negative taxis. Some examples of bacterial taxis include, light (phototaxis), temperature gradients (thermotaxis), and, most commonly, chemical gradients (chemotaxis). Purposeful movement toward a chemical attractant, like a food source, or away from a repellent, like a poisonous chemical, is achieved by increasing the length of runs and decreasing the length of tumbles. When running, flagella rotate in a counterclockwise direction, allowing the bacterial cell to move forward. In a peritrichous bacterium, the flagella are all bundled together in a very streamlined way (Figure 2.18), allowing for efficient movement. When tumbling, flagella are splayed out while rotating in a clockwise direction, creating a looping motion and preventing meaningful forward movement but reorienting the cell toward the direction of the attractant. When an attractant exists, runs and tumbles still occur; however, the length of runs is longer, while the length of the tumbles is reduced, allowing overall movement toward the higher concentration of the attractant. When no chemical gradient exists, the lengths of runs and tumbles are more equal, and overall movement is more random (Figure 2.19).
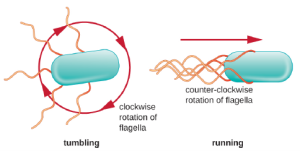
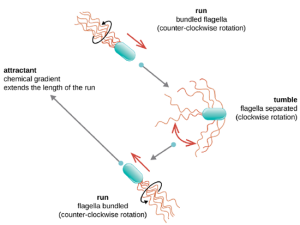
Cell Division: The bacterial cell cycle involves the formation of new cells through the replication of DNA and partitioning of cellular components into two daughter cells. In prokaryotes, reproduction is always asexual, although extensive genetic recombination in the form of horizontal gene transfer takes place. The most common mechanism of cell replication in bacteria is a process called binary fission, which is depicted in Figure 2.20. Before dividing, the cell grows and increases its number of cellular components. Next, the replication of DNA starts at a location on the circular chromosome called the origin of replication, where the chromosome is attached to the inner cell membrane. Replication continues in opposite directions along the chromosome until the terminus is reached.
The center of the enlarged cell constricts until two daughter cells are formed, each offspring receiving a complete copy of the parental genome and a division of the cytoplasm (cytokinesis). New peptidoglygan is formed between the membranes of the new daughter cells forming a structure called a septum. The daughter cells are separated by the division septum, where all of the cells’ outer layers (the cell wall and outer membranes, if present) must be remodeled to complete division.
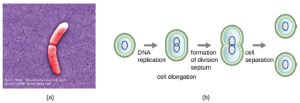
Genetic Variation: Typically, when we consider genetic transfer, we think of vertical gene transfer, the transmission of genetic information from generation to generation. Vertical gene transfer is by far the main mode of transmission of genetic information in all cells. In sexually reproducing organisms, crossing-over events and independent assortment of individual chromosomes during meiosis contribute to genetic diversity in the population. Genetic diversity is also introduced during sexual reproduction, when the genetic information from two parents, each with different complements of genetic information, are combined, producing new combinations of parental genotypes in the diploid offspring. The occurrence of mutations also contributes to genetic diversity in a population. Genetic diversity of offspring is useful in changing or inconsistent environments and may be one reason for the evolutionary success of sexual reproduction.
When prokaryotes and eukaryotes reproduce asexually, they transfer a nearly identical copy of their genetic material to their offspring through vertical gene transfer. Although asexual reproduction produces more offspring more quickly, any benefits of diversity among those offspring are lost. How then do organisms whose dominant reproductive mode is asexual create genetic diversity? In prokaryotes, horizontal gene transfer (HGT), the introduction of genetic material from one organism to another organism within the same generation, is an important way to introduce genetic diversity. HGT allows even distantly related species to share genes, influencing their phenotypes. Horizontal gene transfer in prokaryotes occurs through three primary mechanisms: Transformation, Transduction and Conjugation (Figure 2.21).
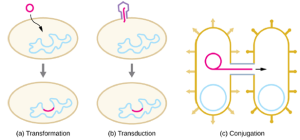
- Transformation: In transformation, the prokaryote takes up naked DNA found in its environment and that is derived from other cells that have lysed on death and released their contents, including their genome, into the environment. If the bacterium incorporates the new DNA into its own genome through recombination, the bacterial cell may gain new phenotypic properties. For example, if a nonpathogenic bacterium takes up DNA for a toxin gene from a pathogen and then incorporates it into its chromosome, it, too, may become pathogenic. Plasmid DNA may also be taken up by competent bacteria and confer new properties to the cell.
In nature, bacterial transformation is an important mechanism for the acquisition of genetic elements encoding virulence factors and antibiotic resistance. Genes encoding resistance to antimicrobial compounds have been shown to be widespread in nature, even in environments not influenced by humans. These genes, which allow microbes living in mixed communities to compete for limited resources, can be transferred within a population by transformation, as well as by the other processes of HGT.
- Transduction: Viruses that infect bacteria (bacteriophages) may also move short pieces of chromosomal DNA from one bacterium to another in a process called transduction. The specific mechanisms of transduction will be discussed in a later unit. Of medical significance, a bacteriophage may carry with it a virulence gene to its new host. Once inserted into the new host’s chromosome, the new host may gain pathogenicity. Several pathogenic bacteria, including Clostridium botulinum (the causative agent of botulism), are virulent because of the introduction of toxin-encoding genes by bacteriophages, affirming the clinical relevance of transduction in the exchange of genes involved in infectious disease.
- Conjugation: In conjugation, DNA is directly transferred from one prokaryote to another by means of a conjugation pilus, which brings the organisms into contact with one another. Conjugation can facilitate the transfer of plasmids, or even the whole bacterial chromosome. Plasmids are an important type of extrachromosomal DNA element in bacteria and, in those cells that harbor them, are considered to be part of the bacterial genome. From a clinical perspective, plasmids often code for genes involved in virulence. For example, genes encoding proteins that make a bacterial cell resistant to a particular antibiotic are encoded on plasmids. Genes required for the production of various toxins and molecules important for colonization during infection may also be found encoded on plasmids.
Unique Characteristics of Eukaryotic Cells
Eukaryotic organisms include protozoans, algae, fungi, plants, and animals. Some eukaryotic cells are independent, single-celled microorganisms, whereas others are part of multicellular organisms. The cells of eukaryotic organisms have several distinguishing characteristics. Above all, eukaryotic cells are defined by the presence of a nucleus surrounded by a complex nuclear membrane. Also, eukaryotic cells are characterized by the presence of membrane-bound organelles in the cytoplasm. Organelles such as mitochondria, the endoplasmic reticulum (ER), Golgi apparatus, lysosomes, and peroxisomes are held in place by the cytoskeleton, an internal network that supports transport of intracellular components and helps maintain cell shape (Figure 2.1). The genome of eukaryotic cells is packaged in multiple, rod-shaped chromosomes as opposed to the single, circular-shaped chromosome that characterizes most prokaryotic cells. Table 2.2 compares the characteristics of eukaryotic cell structures with those of bacteria.
Table 2.2 Summary of Cell Structures
Nucleus: Unlike prokaryotic cells, in which DNA is loosely contained in the nucleoid region, eukaryotic cells possess a nucleus, which is surrounded by a complex nuclear membrane that houses the DNA genome (Figure 2.1). By containing the cell’s DNA, the nucleus ultimately controls all activities of the cell and also serves an essential role in reproduction and heredity. Eukaryotic cells typically have their DNA organized into multiple linear chromosomes. The DNA within the nucleus is highly organized and condensed to fit inside the nucleus, which is accomplished by wrapping the DNA around proteins called histones.
Ribosomes: Ribosomes found in eukaryotic organelles such as mitochondria or chloroplasts have 70S ribosomes—the same size as prokaryotic ribosomes. However, nonorganelle-associated ribosomes in eukaryotic cells are 80S ribosomes, composed of a 40S small subunit and a 60S large subunit. In terms of size and composition, this makes them distinct from the ribosomes of prokaryotic cells.
The two types of nonorganelle-associated eukaryotic ribosomes are defined by their location in the cell: free ribosomes and membrane-bound ribosomes. Free ribosomes are found in the cytoplasm and serve to synthesize water-soluble proteins; membrane-bound ribosomes are found attached to the rough endoplasmic reticulum and make proteins for insertion into the cell membrane or proteins destined for export from the cell. The differences between eukaryotic and prokaryotic ribosomes are clinically relevant because certain antibiotic drugs are designed to target one or the other.
Endomembrane System and other organelles: The endomembrane system, unique to eukaryotic cells, is a series of membranous tubules, sacs, and flattened disks that synthesize many cell components and move materials around within the cell. Because of their larger cell size, eukaryotic cells require this system to transport materials that cannot be dispersed by diffusion alone. The endomembrane system comprises several organelles and connections between them, including the endoplasmic reticulum, Golgi apparatus, lysosomes, and vesicles. Mitochondria and chloroplasts (the organelle responsible for photosynthesis) are also unique to eukaryotic cells and are thought to have arisen from free-living bacteria.
Cytoskeleton: While both prokaryotes and eukaryotes contain cytoskeletons, networks for proteins that provide internal structural support, the cytoskeleton of eukaryotic cells is more advanced, allowing for specialized functions. Temporary extensions of the cytoplasmic membrane called pseudopodia (meaning “false feet”) are produced through modifications of the cytoskeleton, allowing for cells to crawl along substrates. Beyond amoeboid movement, the cytoskeleton is also involved in a variety of other processes in eukaryotic cells, including cytoplasmic streaming (the movement or circulation of cytoplasm within the cell), cleavage furrow formation during cell division, and muscle movement in animals.
Plasma Membrane: The plasma membrane of eukaryotic cells is similar in structure to the prokaryotic plasma membrane in that it is composed mainly of phospholipids forming a bilayer with embedded peripheral and integral proteins. These membrane components move within the plane of the membrane according to the fluid mosaic model. However, unlike the prokaryotic membrane, eukaryotic membranes contain sterols, including cholesterol, that alter membrane fluidity. Additionally, many eukaryotic cells contain some specialized lipids, including sphingolipids, which are thought to play a role in maintaining membrane stability as well as being involved in signal transduction pathways and cell-to-cell communication.
Cell Wall: In addition to a plasma membrane, some eukaryotic cells have a cell wall. Cells of fungi, algae, plants, and even some protozoa have cell walls. Depending upon the type of eukaryotic cell, cell walls can be made of a wide range of materials, including cellulose (fungi and plants); biogenic silica, calcium carbonate, agar, and carrageenan (protozoa and algae); or chitin (fungi). In general, all cell walls provide structural stability for the cell and protection from environmental stresses such as desiccation, changes in osmotic pressure, and traumatic injury.
Extracellular matrix: Cells of animals and some protozoans do not have cell walls to help maintain shape and provide structural stability. Instead, these types of eukaryotic cells produce an extracellular matrix for this purpose. They secrete a sticky mass of carbohydrates and proteins into the spaces between adjacent cells. The extracellular matrix is in some ways analogous to the glycocalyx in prokaryotes. In animal cells, the extracellular matrix allows cells within tissues to withstand external stresses and transmits signals from the outside of the cell to the inside. In addition, a host cell’s extracellular matrix is often the site where microbial pathogens attach themselves to establish infection. For example, Streptococcus pyogenes, the bacterium that causes strep throat and various other infections, binds to fibronectin in the extracellular matrix of the cells lining the oropharynx (upper region of the throat).
Flagella and Cilia: Some eukaryotic cells use flagella for locomotion; however, eukaryotic flagella are structurally distinct from those found in prokaryotic cells. Whereas the prokaryotic flagellum is a stiff, rotating structure, a eukaryotic flagellum is more like a flexible whip composed of nine parallel pairs of microtubules surrounding a central pair of microtubules. The parallel microtubules use motor proteins to move relative to each other, causing the flagellum to bend (Figure 2.22). Cilia (singular: cilium) are a similar external structure found in some eukaryotic cells. Unique to eukaryotes, cilia are shorter than flagella and often cover the entire surface of a cell; however, they are structurally similar to flagella (a 9+2 array of microtubules) and use the same mechanism for movement. Because of their shorter length, cilia use a rapid, flexible, waving motion. In addition to motility, cilia may have other functions such as sweeping particles past or into cells. For example, ciliated protozoans use the sweeping of cilia to move food particles into their mouthparts, and ciliated cells in the mammalian respiratory tract beat in synchrony to sweep mucus and debris up and out of the lungs (Figure 2.22)
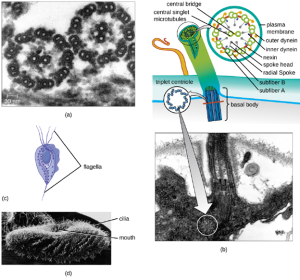
Cell Division: Recall from Biol1203 that eukaryotic cells can undergo mitosis and meiosis. The details of these processes will not be rehashed here but recall that one “turn” or cycle of the cell cycle consists of two general phases: interphase, followed by mitosis and cytokinesis. Interphase is the period of the cell cycle during which the cell is not dividing. The majority of cells are in interphase most of the time. Mitosis is the division of genetic material, during which the cell nucleus breaks down and two new, fully
functional, nuclei are formed. Cytokinesis divides the cytoplasm into two distinctive cells. The purpose of mitosis is creating two identical daughter cells containing the same genetic material. It is used during grow and repair and is used for asexual reproduction. Meiosis is only used for the generation of gametes which are used for sexual reproduction. The purpose of meiosis is to generate non-identical, haploid cells. These cells contain only one copy of each chromosome and the resultant four daughter cells are distinct from each other and to their parental cell, due to the processes of independent assortment and recombination.
Cell Structure | Prokaryotes (Bacteria) | Eukaryotes |
---|---|---|
Size | ~0.5–1 μM | ~5–20 μM |
Surface area-to-volume ratio | High | Low |
Nucleus | No | Yes |
Genome characteristics | • Single chromosome
• Circular • Haploid • Lacks histones |
• Multiple chromosomes
• Linear • Haploid or diploid • Contains histones |
Cell division | Binary Fission | Mitosis, meiosis |
Sexual recombination | None; transfer of DNA only | Involves meiosis |
Plasma membrane composition | Lacks sterols | Contains sterols |
Cell wall composition | • Peptidoglycan
• Occasionally, none |
• Cellulose (plants, some algae)
• Chitin (mollusks, insects crustaceans and fungi) • Silica (some algae) • Most others lack cell walls |
Motility structures | Rigid, spiral flagella composed of flagellin | Flexible flagella and cilia composed of microtubules |
Membrane-bound organelles | Absent | Present |
Ribosomes | 70S in cytoplasm | • 80S free in cytoplasm and bound to the endoplasmic reticulum
• 70S in mitochondria |
Cytoplasmic streaming | Absent | Present |