Unit 2.3 Metabolism
Outline
Learning Objectives
After reading the following, you should be able to:
- Describe metabolism.
- Differentiate the steps involved in aerobic respiration in prokaryotes versus eukaryotes.
- Describe two alternative pathways for glucose metabolism (Entner-Doudoroff pathway and Pentose Phosphate Pathway)
- Describe anaerobic respiration and fermentation and be able to compare the energy yield of these pathways in comparison to aerobic respiration.
- Briefly describe lipid and protein metabolism
- Describe metabolic diversity
The term used to describe all of the chemical reactions inside a cell is metabolism (Figure 2.23). Cellular processes such as the building or breaking down of complex molecules occur through series of stepwise, interconnected chemical reactions called metabolic pathways. Reactions that are spontaneous and release energy are exergonic reactions, whereas endergonic reactions require energy to proceed. The term anabolism refers to those endergonic metabolic pathways involved in biosynthesis, converting simple molecular building blocks into more complex molecules, and fueled by the use of cellular energy. Conversely, the term catabolism refers to exergonic pathways that break down complex molecules into simpler ones. Molecular energy stored in the bonds of complex molecules is released in catabolic pathways and harvested in such a way that it can be used to produce high-energy molecules, which are used to drive anabolic pathways. Thus, in terms of energy and molecules, cells are continually balancing catabolism with anabolism.
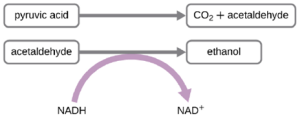
Oxidation and Reduction: The transfer of electrons between molecules is important because most of the energy stored in atoms and used to fuel cell functions is in the form of high-energy electrons. The transfer of energy in the form of electrons allows the cell to transfer and use energy incrementally; that is, in small packages rather than a single, destructive burst. Reactions that remove electrons from donor molecules, leaving them oxidized, are oxidation reactions; those that add electrons to acceptor molecules, leaving them reduced, are reduction reactions. Because electrons can move from one molecule to another, oxidation and reduction occur in tandem. These pairs of reactions are called oxidation-reduction reactions, or redox reactions.
Energy Carriers: NAD+, NADP+, FAD, and ATP: The energy released from the breakdown of the chemical bonds within nutrients can be stored either through the reduction of electron carriers or in the bonds of adenosine triphosphate (ATP). In living systems, a small class of compounds functions as mobile electron carriers, molecules that bind to and shuttle high-energy electrons between compounds in pathways. The principal electron carriers we will consider originate from the B vitamin group and are derivatives of nucleotides; they are nicotinamide adenine dinucleotide (NAD+/NADH), nicotine adenine dinucleotide phosphate (NADP+/NADPH), and flavin adenine dinucleotide (FAD/FADH2). Both NAD+/NADH and FAD/FADH2 are extensively used in energy extraction from sugars during catabolism, whereas NADP+/NADPH plays an important role in anabolic reactions and photosynthesis.
A living cell must be able to handle the energy released during catabolism in a way that enables the cell to store energy safely and release it for use only as needed. Living cells accomplish this by using the compound adenosine triphosphate (ATP). ATP is often called the “energy currency” of the cell, and, like currency, this versatile compound can be used to fill any energy need of the cell. At the heart of ATP is a molecule of adenosine monophosphate (AMP), which is composed of an adenine molecule bonded to a ribose molecule and a single phosphate group. Ribose is a five-carbon sugar found in RNA, and AMP is one of the nucleotides in RNA. The addition of a second phosphate group to this core molecule results in the formation of adenosine diphosphate (ADP); the addition of a third phosphate group forms ATP (Figure 2.24). Adding a phosphate group to a molecule, a process called phosphorylation, requires energy. Removing a phosphate group, releases energy that can be used to drive catabolic processes.
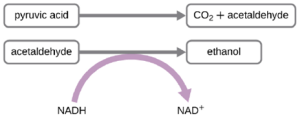
Glycolysis: For bacteria, eukaryotes, and most archaea, glycolysis is the most common pathway for the catabolism of glucose; it produces energy, reduced electron carriers, and precursor molecules for cellular metabolism. Every living organism carries out some form of glycolysis, suggesting this mechanism is an ancient universal metabolic process. The process itself does not use oxygen; however, glycolysis can be coupled with additional metabolic processes that are either aerobic or anaerobic. Glycolysis takes place in the cytoplasm of prokaryotic and eukaryotic cells. It begins with a single six-carbon glucose molecule and ends with two molecules of a three-carbon sugar called pyruvate. Pyruvate may be broken down further after glycolysis to harness more energy through aerobic or anaerobic respiration, but many organisms, including many microbes, may be unable to respire; for these organisms, glycolysis may be their only source of generating ATP.
The first part of the pathway, called the energy investment phase, uses energy from two ATP molecules to modify a glucose molecule so that the six-carbon sugar molecule can be split evenly into two phosphorylated three-carbon molecules called glyceraldehyde 3-phosphate (G3P). The second part of the pathway, called the energy payoff phase, extracts energy by oxidizing G3P to pyruvate, producing four ATP molecules and reducing two molecules of NAD+ to two molecules of NADH, using electrons that originated from glucose. The ATP molecules produced during the energy payoff phase of glycolysis are formed by substrate-level phosphorylation, one of two mechanisms for producing ATP. In substrate-level phosphorylation, a phosphate group is removed from an organic molecule and is directly transferred to an available ADP molecule, producing ATP.
Overall, in this process of glycolysis, the net gain from the breakdown of a single glucose molecule is:
- two ATP molecules
- two NADH molecule, and
- two pyruvic acid molecules.
Other Glycolytic Pathways: While glycolysis is the most common process from the breakdown of glucose, some prokaryotes use alternative glycolytic pathways. One important alternative is the
Entner-Doudoroff pathway. The Entner-Doudoroff pathway also generates pyruvic acid, but does so in a less efficient fashion than glycolysis. Some bacteria, including the opportunistic gram-negative pathogen Pseudomonas aeruginosa, contain only the Entner-Doudoroff pathway for the breakdown of glucose, other bacteria, like E. coli, have the ability to use either the Entner-Doudoroff pathway or the glycolysis pathway.
Overall, in the Entner-Doudoroff pathway, the net gain from the breakdown of a single glucose molecule is:
- one ATP molecule
- one NADPH molecule
- one NADH molecule, and
- two pyruvic acid molecules.
A third type of glycolytic pathway that occurs in all cells, which is quite different from the previous two pathways, is the pentose phosphate pathway. Evidence suggests that the PPP may be the most ancient universal glycolytic pathway. The intermediates from the PPP are used for the biosynthesis of nucleotides and amino acids. Therefore, this glycolytic pathway may be favored when the cell has need for nucleic acid and/or protein synthesis, respectively. Bacteria that use the pentose phosphate pathway include E. coli and Enterococcus faecalis.
Overall, in the pentose phosphate pathway, the net gain from the breakdown of a single glucose molecule is:
- two NADPH molecules, and
- one ribose molecule
Cellular respiration: After glucose has been broken down into pyruvic acid, it can be channeled into either fermentation pathways or cellular respiration. Cellular respiration is and ATP-generating process which oxidizes molecules and the terminal electron acceptor comes from outside the cell and is usually an inorganic molecule. There are two types of respiration, aerobic respiration where the terminal electron acceptor is a molecule of oxygen and anaerobic respiration where the terminal electron acceptor is an inorganic molecule other than oxygen. Organisms can be classified by the type of cellular respiration they use, aerobes use aerobic respiration and often die in the absence of oxygen and anaerobes, which grow in the absence oxygen and can even die in its presence.
- Aerobic respiration: Glycolysis produces pyruvate, which can be further oxidized to capture more energy. For pyruvate to enter the next oxidative pathway, it must first be decarboxylated to form acetyl-coA. The transition reaction occurs in the mitochondrial matrix of eukaryotes; in prokaryotes, it occurs in the cytoplasm because prokaryotes lack membrane-enclosed organelles. The acetyl-coA molecules then enter the Krebs cycle (also known as the TCA or citric acid cycle). The Krebs cycle transfers remaining electrons from the acetyl group produced during the transition reaction to electron carrier molecules, thus reducing them. The Krebs cycle also occurs in the cytoplasm of prokaryotes along with glycolysis and the transition reaction, but it takes place in the mitochondrial matrix of eukaryotic cells where the transition reaction also occurs. Unlike glycolysis, the Krebs cycle is a closed loop: The last part of the pathway regenerates the compound used in the first step (Figure 2.25). As one turn of the cycle returns to the starting point of the four-carbon intermediate, the cycle produces two CO2 molecules, one ATP molecule produced by substrate-level phosphorylation, and three molecules of NADH and one of FADH2. Although many organisms use the Krebs cycle as described as part of glucose metabolism, several of the intermediate compounds in the Krebs cycle can be used in synthesizing a wide variety of important cellular molecules, including amino acids, chlorophylls, fatty acids, and nucleotides; therefore, the cycle is both anabolic and catabolic.
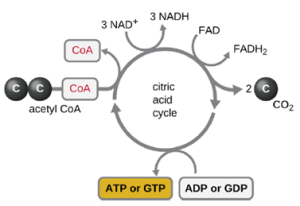
We have just discussed two pathways in glucose catabolism—glycolysis and the Krebs cycle—that generate ATP by substrate-level phosphorylation. Most ATP, however, is generated during a separate process called oxidative phosphorylation. The process begins when electrons from NADH and FADH2 generated in the previous steps, are transferred through a series of chemical reactions through the electron transport chain, to a final inorganic electron acceptor, oxygen in the case of aerobic respiration. These electron transfers take place on the inner part of the cell membrane of prokaryotic cells or in specialized protein complexes in the inner membrane of the mitochondria of eukaryotic cells. The energy of the electrons is harvested to generate an electrochemical gradient across the membrane, which is used to make ATP by oxidative phosphorylation (Figure 2.26).
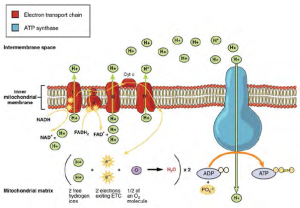
The number of ATP molecules generated from the catabolism of glucose varies. For example, the number of hydrogen ions that the electron transport system complexes can pump through the membrane varies between different species of organisms. In aerobic respiration in mitochondria, the passage of electrons from one molecule of NADH generates enough proton motive force to make three ATP molecules by oxidative phosphorylation, whereas the passage of electrons from one molecule of FADH2 generates enough proton motive force to make only two ATP molecules. Based on those numbers and taking into account the ATP produced by substrate-level phosphorylation in earlier steps, the maximal number of ATP that can be produced by aerobic respiration is 32. This is rarely the case, due to inefficiencies in the processes, but prokaryotic cells are able to produce closer to the maximum. Since all their reactions occur in the cytoplasm, they don’t have to expend energy to transport intermediates from the cytoplasm into the mitochondria, as is the case in eukaryotic cells.
- Anaerobic respiration: Prokaryotes that perform anaerobic respiration use the same processes as those that use aerobic respiration. Pyruvate that is produced from glycolysis enters into the Krebs cycle, which produced NADH and FADH2 which then moves to the electron transport chain to generated ATP via oxidative phosphorylation. Whereas in aerobic respiration the terminal electron acceptor in the chain is always oxygen, which is cleaved to form 2 water molecules, there are different types of anaerobic respiration each using a different inorganic molecule as the terminal electron acceptor.
Some common molecules include:
- Nitrate which can be reduced to nitrous oxide or nitrogen gas
- Sulfate which can be reduced to form hydrogen sulfide
- Carbonate which can be reduced to form methane
While the basic principles are the same, there are some key differences in how these processes work in anaerobes compared to aerobes. First, not all parts of the Krebs cycle can function in the absence of oxygen, so anaerobic bacteria use modified cycles. Second, anaerobic respirers use altered electron transport chain protein carriers, including distinct complexes for electron transfer specific to their final electron acceptors, and smaller proton gradients are generated from these electron transfer systems. The net effect of these differences is that ATP yield from anaerobic respiration is never as high as in aerobic respiration and so, anaerobes tend to grow more slowly than aerobes.
Fermentation: Many cells are unable to carry out respiration because of one or more of the following circumstances:
- The cell lacks a sufficient amount of any appropriate, inorganic, final electron acceptor to carry out cellular respiration.
- The cell lacks genes to make appropriate complexes and electron carriers in the electron transport system.
- The cell lacks genes to make one or more enzymes in the Krebs cycle.
Whereas lack of an appropriate inorganic final electron acceptor is environmentally dependent, the other two conditions are genetically determined. Thus, many prokaryotes, including members of the clinically important genus Streptococcus, are permanently incapable of respiration, even in the presence of oxygen. Conversely, many prokaryotes are facultative, meaning that, should the environmental conditions change to provide an appropriate inorganic final electron acceptor for respiration, organisms containing all the genes required to do so will switch to cellular respiration for glucose metabolism because respiration allows for much greater ATP production per glucose molecule.
Glycolysis is independent of cellular respiration, but requires NAD+ for the production of NADH. In the absence of NAD+, glycolysis stops. In respiration, this NAD+ is generated by the electron transport chain. In the absence of respiration, NADH must be reoxidized to NAD+ by other means for reuse as an electron carrier for glycolysis, the cell’s only mechanism for producing any ATP, to continue. The reoxidation is facilitated by fermentation pathways. Fermentation does not involve an electron transport system and does not directly produce any additional ATP beyond that produced during glycolysis by substrate-level phosphorylation. Organisms carrying out fermentation, called fermenters, produce a maximum of two ATP molecules per glucose during glycolysis. Table 2.3 compares the final electron acceptors and methods of ATP synthesis in aerobic respiration, anaerobic respiration, and fermentation. Note that the number of ATP molecules shown is for glycolysis. The number of ATP molecules made by substrate-level phosphorylation (SLP) versus oxidative phosphorylation (OP) are indicated.
Fermentation by some bacteria, like those in yogurt and other soured food products, and by animals in muscles during oxygen depletion, is lactic acid fermentation. The chemical reaction of lactic acid fermentation is as follows:
Pyruvate + NADH ↔ lactic acid + NAD+
Bacteria of several gram-positive genera, including Lactobacillus, Leuconostoc, and Streptococcus, are collectively known as the lactic acid bacteria (LAB), and various strains are important in food production. During yogurt and cheese production, the highly acidic environment generated by lactic acid fermentation denatures proteins contained in milk, causing it to solidify. Lactic acid bacteria are also important medically. The production of low pH environments within the body inhibits the establishment and growth of pathogens in these areas. For example, the vaginal microbiota is composed largely of lactic acid bacteria, but when these bacteria are reduced, yeast can proliferate, causing a yeast infection.
Type of Metabolism | Example Species | Terminal Electron Acceptor | Pathways Involved in ATP Synthesis (Type of Phosphorylation) | Maximum Yield of ATP Molecules |
---|---|---|---|---|
Aerobic Respiration | Pseudomonas aeruginosa | Oxygen | Glycolysis (SLP)
Krebs cycle (SLP) ETC and chemiosmosis (OP) Total |
2 2 28 32
|
Anaerobic Respiration | Paracoccus denitrificans | Nitrate, Sulfate, Carbonate, and other inorganics | Glycolysis (SLP)
Krebs cycle (SLP) ETC and chemiosmosis (OP) Total |
2 2 1-26 5-30 |
Fermentation | Candida albicans | Pyruvic acid, acetylaldehyde and other organics | Glycolysis (SLP)
Fermentation Total |
2 0 2 |
Another familiar fermentation process is alcohol fermentation, which produces ethanol. The ethanol fermentation reaction is shown in Figure 2.27. In the first reaction, the enzyme pyruvate decarboxylase removes a carboxyl group from pyruvate, releasing CO2 gas while producing the two-carbon molecule acetaldehyde. The second reaction, catalyzed by the enzyme alcohol dehydrogenase, transfers an electron from NADH to acetaldehyde, producing ethanol and NAD+. The ethanol fermentation of pyruvate by the yeast Saccharomyces cerevisiae is used in the production of alcoholic beverages and also makes bread products rise due to CO2 production.
Beyond lactic acid fermentation and alcohol fermentation, many other fermentation methods occur in prokaryotes, all for the purpose of ensuring an adequate supply of NAD+ for glycolysis (Table 8.3). Without these pathways, glycolysis would not occur and no ATP would be harvested from the breakdown of glucose. It should be noted that most forms of fermentation besides homolactic fermentation produce gas, commonly CO2 and/or hydrogen gas. Many of these different types of fermentation pathways are also used in food production and each results in the production of different organic acids, contributing to the unique flavor of a particular fermented food product. The propionic acid produced during propionic acid fermentation contributes to the distinctive flavor of Swiss cheese, for example.
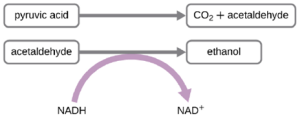
Fermentation products are used in the laboratory to differentiate various bacteria for diagnostic purposes. For example, enteric bacteria are known for their ability to perform mixed acid fermentation, reducing the pH, which can be detected using a pH indicator. Microbes can also be differentiated according to the substrates they can ferment. For example, E. coli can ferment lactose, forming gas, whereas some of its close gram-negative relatives cannot. The ability to ferment the sugar alcohol sorbitol is used to identify the pathogenic enterohemorrhagic O157:H7 strain of E. coli because, unlike other E. coli strains, it is unable to ferment sorbitol. Last, mannitol fermentation differentiates the mannitol-fermenting Staphylococcus aureus from other non–mannitol-fermenting staphylococci.
Protein and Lipid Catabolism: Certain bacteria are able to digest proteins and fats. Recall that triglycerides are a form of long-term energy storage in animals. They are made of glycerol and three fatty acids. Phospholipids compose the cell and organelle membranes of all organisms except the archaea. Triglycerides and phospholipids are broken down first by releasing fatty acid chains
(and/or the phosphorylated head group, in the case of phospholipids) from the three-carbon glycerol backbone. The reactions breaking down triglycerides are catalyzed by lipases and those involving phospholipids are catalyzed by phospholipases. These enzymes are secreted outside of the microbes and function extracellularly. These enzymes also contribute to the virulence of certain microbes, such as the bacterium Staphylococcus aureus and the fungus Cryptococcus neoformans. These microbes use phospholipases to destroy lipids and phospholipids in host cells and then use the catabolic products for energy. The digested products are then absorbed and digested by similar processes as in eukaryotic cells, such as β-oxidation for the digestion of fatty acids.
The digestion of proteins also begins outside the cell, as they are too large to cross the bacterial membrane. Digestion begins with the secretion of proteases and peptidases that break the proteins down into amino acids that then cross the plasma membrane. Some clinically important pathogens can be identified by their ability to produce a specific type of extracellular protease. For example, the production of the extracellular protease gelatinase by members of the genera Proteus and Serratia can be used to distinguish them from other gram-negative enteric bacteria. Once inside the cell, further breakdown of amino acids occurs in a similar fashion as in eukaryotic cells. They first undergo deamination, removing the amino group. This amino group is converted into ammonia and excreted from the cell as a waste product. (In humans, is usually converted into urea, as ammonia is fairly toxic) The remaining organic acid then enters into the Krebs cycle.
Metabolic Diversity: Organisms can be identified according to the source of carbon they use for metabolism as well as their energy source. The prefixes auto- (“self”) and hetero- (“other”) refer to the origins of the carbon sources various organisms can use. Organisms that convert inorganic carbon dioxide (CO2) into organic carbon compounds are autotrophs. Plants and cyanobacteria are well-known examples of autotrophs. Conversely, heterotrophs rely on more complex organic carbon compounds as nutrients; these are provided to them initially by autotrophs. Many organisms, ranging from humans to many prokaryotes, including the well-studied Escherichia coli, are heterotrophic.
Organisms can also be identified by the energy source they use. All energy is derived from the transfer of electrons, but the source of electrons differs between various types of organisms. The prefixes photo- (“light”) and chemo- (“chemical”) refer to the energy sources that various organisms use. Those that get their energy for electron transfer from light are phototrophs, whereas chemotrophs obtain energy for electron transfer by breaking chemical bonds. There are two types of chemotrophs: organotrophs and lithotrophs. Organotrophs, including humans, fungi, and many prokaryotes, are chemotrophs that obtain energy from organic compounds. Lithotrophs (“litho” means “rock”) are chemotrophs that get energy from inorganic compounds, including hydrogen sulfide (H2S) and reduced iron. Lithotrophy is unique to the microbial world.
The strategies used to obtain both carbon and energy can be combined for the classification of organisms according to nutritional type. Most organisms are chemoheterotrophs because they use organic molecules as both their electron and carbon sources. Table 2.4 summarizes this and the other classifications.
Classifications | Energy Source | Carbon Source | Examples | |
---|---|---|---|---|
Chemotrophs | Chemoautotrophs | Chemical | Inorganic | Hydrogen, sulfur, iron, nitrogen, and carbon monoxide oxidizing bacteria |
Chemoheterotrophs | Chemical | Organic compounds | All animals, most fungi, protozoa and bacteria | |
Phototrophs | Photoautotrophs | Light | Inorganic | All plants, algae, cyanobacteria, and green and purple sulfur bacteria |
Photoheterotrophs | Light | Organic compounds | Green and purple nonsulfur bacteria, heliobacteria |