Unit 5.1: Bacterial Growth
Learning Objectives
After reading the following, you should be able to:
- Describe the physical (temperature, pH and osmotic pressure), and chemical requirements for microbial growth.
- Describe how reactive oxygen species (ROS) can affect microorganisms and how microorganisms can overcome ROS.
- Describe biofilms, how they are formed, their benefits to microbes, and why they are a concern in the medical field.
When we are talking about microbial growth, we are talking about an increase in the number of cells in a population not about an increase in the size of the microbial cells themselves. Several factors are required for proper growth and they can be separated into two main categories; Physical factors, which includes things like temperature, pH, and osmotic pressure, and chemical factors, which includes things like carbon, nitrogen, phosphorus, sulfur (as well as other trace elements), oxygen and organic growth factors.
Temperature: When the exploration of Lake Whillans started in Antarctica, researchers did not expect to find much life. Constant subzero temperatures and lack of obvious sources of nutrients did not seem to be conditions that would support a thriving ecosystem. To their surprise, the samples retrieved from the lake showed abundant microbial life. In a different but equally harsh setting, bacteria grow at the bottom of the ocean in sea vents (Figure 5.1), where temperatures can reach 340 °C (700 °F).
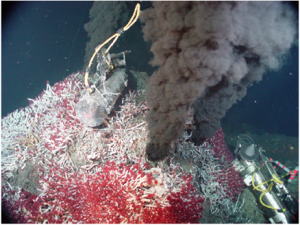
Microbes can be roughly classified according to the range of temperature at which they can grow. The growth rates are the highest at the optimum growth temperature for the organism. The lowest temperature at which the organism can survive and replicate is its minimum growth temperature. The highest temperature at which growth can occur is its maximum growth temperature. The following ranges of permissive growth temperatures are approximate only and can vary according to other environmental factors. It is also important to note, that in general, metabolic processes are faster at higher temperatures, so, while there are exceptions, bacteria with higher optimal growth temperatures will grow faster than those species with lower optimal growth temperatures.
Organisms categorized as mesophiles (“middle loving”) are adapted to moderate temperatures, with optimal growth temperatures ranging from room temperature (about 20 °C) to about 45 °C. As would be expected from the core temperature of the human body, 37 °C (98.6 °F), normal human microbiota and pathogens (e.g., E. coli, Salmonella spp., and Lactobacillus spp.) are mesophiles.
Organisms called psychrotrophs, also known as psychrotolerant, prefer cooler environments, from a high temperature of 25 °C to refrigeration temperature about 4 °C. They are found in many natural environments in temperate climates. They are also responsible for the spoilage of refrigerated food.
The organisms retrieved from arctic lakes such as Lake Whillans are considered extreme psychrophiles (cold loving). Psychrophiles are microorganisms that can grow at 0 °C and below, have an optimum growth temperature close to 15 °C, and usually do not survive at temperatures above 20 °C. They are found in permanently cold environments such as the deep waters of the oceans. Because they are active at low temperature, psychrophiles and psychrotrophs are important decomposers in cold climates.
Organisms that grow at optimum temperatures of 50 °C to a maximum of 80 °C are called thermophiles (“heat loving”). They do not multiply at room temperature. Thermophiles are widely distributed in hot springs, geothermal soils, and manmade environments such as garden compost piles where the microbes break down kitchen scraps and vegetal material. Examples of thermophiles include Thermus aquaticus and Geobacillus spp. Higher up on the extreme temperature scale we find the hyperthermophiles, which are characterized by growth ranges from 80 °C to a maximum of 110 °C, with some extreme examples that survive temperatures above 121 °C, the average temperature of an autoclave. The hydrothermal vents at the bottom of the ocean are a prime example of extreme environments, with temperatures reaching an estimated 340 °C (Figure 5.1). Microbes isolated from the vents achieve optimal growth at temperatures higher than 100 °C. Noteworthy examples are Pyrobolus and Pyrodictium, archaea that grow at 105 °C and survive autoclaving. Figure 5.2 shows the typical skewed curves of temperature-dependent growth for the categories of microorganisms we have discussed.
pH: Yogurt, pickles, sauerkraut, and lime-seasoned dishes all owe their tangy taste to a high acid content. Recall that acidity is a function of the concentration of hydrogen ions [H+] and is measured as pH. Environments with pH values below 7.0 are considered acidic, whereas those with pH values above 7.0 are considered basic. The optimum growth pH is the most favorable pH for the growth of an organism. The lowest pH value that an organism can tolerate is called the minimum growth pH and the highest pH is the maximum growth pH. These values can cover a wide range, which is important for the preservation of food and to microorganisms’ survival in the stomach. For example, the optimum growth pH of Salmonella spp. is 7.0–7.5, but the minimum growth pH is closer to 4.2.
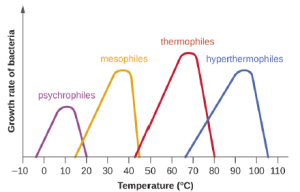
Most bacteria are neutrophiles, meaning they grow optimally at a pH within one or two pH units of the neutral pH of 7 (see Figure 5.3). Most familiar bacteria, like Escherichia coli, staphylococci, and Salmonella spp. are neutrophiles and do not fare well in the acidic pH of the stomach. However, there are pathogenic strains of E. coli, S. typhi, and other species of intestinal pathogens that are much more resistant to stomach acid. In comparison, fungi thrive at slightly acidic pH values of 5.0–6.0.
Microorganisms that grow optimally at pH less than 5.55 are called acidophiles. For example, the sulfur-oxidizing Sulfolobus spp. isolated from sulfur mud fields and hot springs in Yellowstone National Park are extreme acidophiles. Lactobacillus bacteria, which are an important part of the normal microbiota of the vagina, can tolerate acidic environments at pH values 3.5–6.8 and also contribute to the acidity of the vagina (pH of 4, except at the onset of menstruation) through their metabolic production of lactic acid. The vagina’s acidity plays an important role in inhibiting other microbes that are less tolerant of acidity.
At the other end of the spectrum are alkaliphiles, microorganisms that grow best at pH between 8.0 and 10.5. Vibrio cholerae, the pathogenic agent of cholera, grows best at the slightly basic pH of 8.0; it can survive pH values of 11.0, but is inactivated by the acid of the stomach. When it comes to survival at high pH, the bright pink Archaean Natronobacterium, found in the soda lakes of the African Rift Valley, may hold the record at a pH of 10.5 (Figure 5.4).
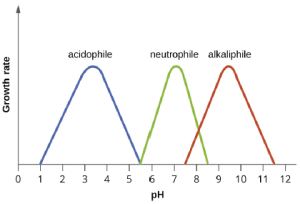
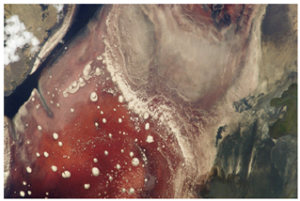
Osmotic Pressure: Recall that osmotic pressure occurs because of differences in the concentration of solutes on opposing sides of a semipermeable membrane. Water is able to pass through a semipermeable membrane, but solutes (dissolved molecules like salts, sugars, and other compounds) cannot. When the concentration of solutes is greater on one side of the membrane, water diffuses across the membrane from the side with the lower concentration (more water) to the side with the higher concentration (less water) until the concentrations on both sides become equal. This diffusion of water is called osmosis, and it can cause extreme osmotic pressure on a cell when its external environment changes.
The external environment of a cell can be described as an isotonic, hypertonic, or hypotonic medium. In an isotonic medium, the solute concentrations inside and outside the cell are approximately equal, so there is no net movement of water across the cell membrane. In a hypertonic medium, the solute concentration outside the cell exceeds that inside the cell, so water diffuses out of the cell and into the external medium. In a hypotonic medium, the solute concentration inside the cell exceeds that outside of the cell, so water will move by osmosis into the cell. This causes the cell to swell and potentially lyse, or burst.
The degree to which a particular cell is able to withstand changes in osmotic pressure is called tonicity. Cells that have a cell wall are better able to withstand subtle changes in osmotic pressure and maintain their shape. In hypertonic environments, cells that lack a cell wall can become dehydrated, causing crenation, or shriveling of the cell; the plasma membrane contracts and appears scalloped or notched (Figure 5.5). By contrast, cells that possess a cell wall undergo plasmolysis rather than crenation. In plasmolysis, the plasma membrane contracts and detaches from the cell wall, and there is a decrease in interior volume, but the cell wall remains intact, thus allowing the cell to maintain some shape and integrity for a period of time (Figure 5.6). Likewise, cells that lack a cell wall are more prone to lysis in hypotonic environments. The presence of a cell wall allows the cell to maintain its shape and integrity for a longer time before lysing (Figure 5.6).
Most natural environments tend to have lower solute concentrations than the cytoplasm of most microorganisms. Rigid cell walls protect the cells from bursting in a dilute environment. Not much protection is available against high osmotic pressure. In this case, water, following its concentration gradient, flows out of the cell. This results in plasmolysis (the shrinking of the protoplasm away from the intact cell wall) and cell death. This fact explains why brines and layering meat and fish in salt are time-honored methods of preserving food. Microorganisms called halophiles (“salt loving”) actually require high salt concentrations for growth. Others are halotolerant meaning that they do not need high concentrations of salt for growth, but they will survive and divide in the presence of high salt. Not surprisingly, the staphylococci, micrococci, and corynebacteria that colonize our skin tolerate salt in their environment. Halotolerant pathogens are an important cause of food-borne illnesses because they survive and multiply in salty food. For example, the halotolerant bacteria S. aureus, Bacillus cereus, and V. cholerae produce dangerous enterotoxins and are major causes of food poisoning.
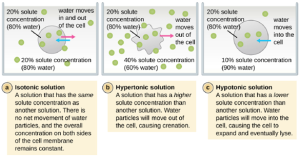
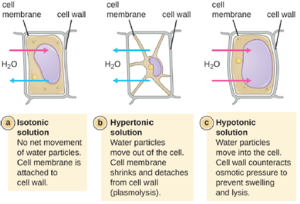
Elements of living cells: The most abundant element in cells is hydrogen (H), followed by carbon (C), oxygen (O), nitrogen (N), phosphorous (P), and sulfur (S). We call these elements macronutrients, and they account for about 99% of the dry weight of cells. Some elements, such as sodium (Na), potassium (K), magnesium (Mg), zinc (Zn), iron (Fe), calcium (Ca), molybdenum (Mo), copper (Cu), cobalt (Co), manganese (Mn), or vanadium (Va), are required by some cells in very small amounts and are called micronutrients or trace elements. All of these elements are essential to the function of many biochemical reactions, and, therefore, are essential to life.
- Carbon: Carbon is the structural backbone of organic molecules. Heterotrophic organisms obtain their carbon from the molecules they ingest, whereas autotrophs obtain their carbon from CO2.
- N, S, P: These elements are key components of nucleic acids and proteins. About 14% of the dry weight of a bacterial cell is nitrogen, with the other two elements accounting for another 4%. These elements are also a key component of fertilizer, which can get into aquatic ecosystems causing blooms of algae and bacteria. Cyanobacteria, which can form blooms in marine and freshwater ecosystems, produce toxins called microcystins, which can cause allergic reactions and liver damage when ingested in drinking water or during swimming. Recurring cyanobacterial algal blooms in Lake Erie (Figure 5.7) have forced municipalities to issue drinking water bans for days at a time because of unacceptable toxin levels.
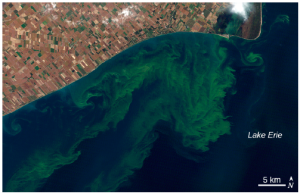
Oxygen and its Reactive Byproducts: Recall that organism can be categorized based on their use and tolerance of oxygen (See Unit 4). Oxygen can be necessary for life or a deadly poison, due to the generation of reactive oxygen species. Aerobic respiration constantly generates reactive oxygen species (ROS), byproducts that must be detoxified. Even organisms that do not use aerobic respiration need some way to break down some of the ROS that may form from atmospheric oxygen. Three main enzymes break down those toxic byproducts: superoxide dismutase, peroxidase, and catalase. Each one catalyzes a different reaction. Reactions of type seen in Reaction 1 are catalyzed by peroxidases.
(1) 2H+ + H2O2 → 2H2O
In these reactions, an electron donor (reduced compound; e.g., NADH) oxidizes hydrogen peroxide, or other peroxides, to water. The enzymes play an important role by limiting the damage caused by peroxidation of membrane lipids. Reaction 2 is mediated by the enzyme superoxide dismutase (SOD) and breaks down the powerful superoxide anions that are generated by aerobic metabolism when oxygen molecules are split:
(2) 2O2− + 2H+ → H2O2 + O2
The enzyme catalase converts hydrogen peroxide to water and oxygen as shown in Reaction 3.
(3) 2H2O2 → 2H2O + O2
Obligate aerobes and facultative anaerobes contain all these enzymes and so are able to survive in the presence of oxygen and use it in their metabolism. Obligate anaerobes lack any of the enzymes and thus cannot survive in the presence of oxygen due to the cellular damage caused by ROSs. Aerotolerant anaerobes have only SOD, so they are able to process the more dangerous superoxide radicals but not hydrogen peroxide. They can survive in the presence of oxygen, but don’t use it in for their metabolism. Microaerophiles contain these enzymes, they work less efficiently than those in obligate aerobes and facultative anaerobes. Because of this, they can only tolerate low concentrations of oxygen, at higher concentrations, their enzymes can’t keep up and the reactive oxygen species accumulate, killing the cells.
Because not all bacterial species contain these enzymes, we can use them as a diagnostic. One such test is the catalase test, which detects the presence of the enzyme catalase. It a useful and rapid test to distinguish streptococci, which are aerotolerant and do not possess catalase, from staphylococci, which are facultative anaerobes. A sample of culture rapidly mixed in a drop of 3% hydrogen peroxide will release bubbles, as oxygen is produced, if the culture is catalase positive (Figure 5.8).
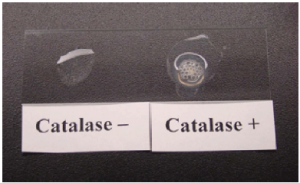
Organic Growth Factors: Just like in humans, certain microbes have essential molecules that they cannot produce themselves and must obtain from their diet. These can be vitamins essential for metabolism, such as those that serve as the precursors to NADH and FADH2, or other molecules, such as amino acids, lipids or nucleotides. The specific organic molecules required will vary from species to species.
Biofilms: In nature, microorganisms grow mainly in biofilms, complex and dynamic ecosystems that form on a variety of environmental surfaces, from industrial conduits and water treatment pipelines to rocks in river beds. Biofilms are not restricted to solid surface substrates, however. Almost any surface in a liquid environment containing some minimal nutrients will eventually develop a biofilm. Microbial mats that float on water, for example, are biofilms that contain large populations of photosynthetic microorganisms. Biofilms found in the human mouth may contain hundreds of bacterial species. Regardless of the environment where they occur, biofilms are not random collections of microorganisms; rather, they are highly structured, slime covered, communities that provide a selective advantage to their constituent microorganisms.
Free-floating microbial cells that live in an aquatic environment are called planktonic cells. The formation of a biofilm essentially involves the attachment of planktonic cells to a substrate, where they become sessile (attached to a surface). This occurs in stages, as depicted in Figure 5.9. The first stage involves the attachment of planktonic cells to a surface coated with a conditioning film of organic material. At this point, attachment to the substrate is reversible, but as cells express new phenotypes that facilitate the formation of extracellular polymeric substances (EPS), through the secretion of carbohydrates and glycoproteins, they transition from a planktonic to a sessile lifestyle. The biofilm develops characteristic structures, including an extensive matrix and water channels. Appendages such as fimbriae, pili, and flagella interact with the extracellular slime, contributing to the recruitment of more members to the growing community. In the last stage of the biofilm life cycle, cells on the periphery of the biofilm revert to a planktonic lifestyle, sloughing off the mature biofilm to colonize new sites. This stage is referred to as dispersal.
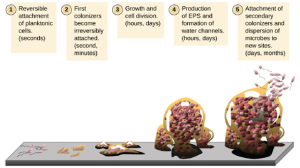
Biofilms are very beneficial to the organisms that reside in them. Living in large numbers provides protection from predation and the presence of the extracellular polymeric substances (slime) covering it, protects all the inhabitants from desiccation, immune attacks and antimicrobial substances such as disinfectants and antibiotics. Within a biofilm, different species of microorganisms establish metabolic collaborations in which the waste product of one organism becomes the nutrient for another. For example, aerobic microorganisms consume oxygen, creating anaerobic regions that promote the growth of anaerobes. This occurs in many polymicrobial infections that involve both aerobic and anaerobic pathogens. Living in close contact with many other bacteria facilitates DNA exchange through transformation, transduction and conjugation. Biofilms also facilitate communication between microbes via a process known as quorum sensing. Quorum sensing—which can occur between cells of different species within a biofilm—enables microorganisms to detect their cell density through the release and binding of small, diffusible molecules and coordinate their activities in response to environmental stimuli. This allows for biofilms to cooperate to accomplish tasks that no single bacteria species could, such as the case in decomposition.
While biofilms provide many benefits to their inhabitants they can be problematic to humans. The human body harbors many types of biofilms, some beneficial and some harmful. For example, the layers of normal microbiota lining the intestinal and respiratory mucosa play a role in warding off infections by pathogens. However, other biofilms in the body can have a detrimental effect on health. For example, the plaque that forms on teeth is a biofilm that can contribute to dental and periodontal disease. Biofilms can also form in wounds, sometimes causing serious infections that can spread. The bacterium Pseudomonas aeruginosa often colonizes biofilms in the airways of patients with cystic fibrosis, causing chronic and sometimes fatal infections of the lungs. Biofilms can also form on medical devices used in or on the body, causing infections in patients with in-dwelling catheters, artificial joints, or contact lenses (Figure 5.10). Once established, biofilms are almost impossible to remove.
Pathogens embedded within biofilms exhibit a higher resistance to antibiotics and other antimicrobial substances, than their free-floating counterparts. Several hypotheses have been proposed to explain why. Cells in the deep layers of a biofilm are metabolically inactive and may be less susceptible to the action of antibiotics that disrupt metabolic activities. The EPS slime coating may also slow the diffusion of antibiotics and antiseptics, preventing them from reaching cells in the deeper layers of the biofilm. Finally, as mentioned above, biofilms provide an ideal environment for the exchange of extrachromosomal DNA, which often includes genes that confer antibiotic resistance.
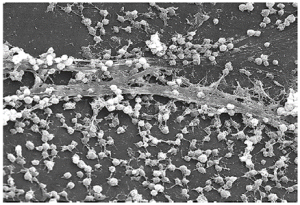