Unit 12: Human Genetics
Unit Outline
Part 1: Cell Growth and Division
Part 2: Patterns of Inheritance
- From Genotype to Phenotype
- Mendel’s Theory of Inheritance
- Autosomal Dominant Inheritance
- Autosomal Recessive Inheritance
- X-linked Dominant or Recessive Inheritance
- Mutations
- Chromosomal Disorders
- Detecting Genetic Disorders
Practice Questions
Learning Objectives
At the end of this unit, you should be able to:
I. Distinguish between significant related genetic terms.
II. Describe the karyotype of a normal human female and the karyotype of a normal human male.
III. Describe the processes of mitosis and meiosis.
IV. Describe the effects of nondisjunction on the chromosome complement of gametes.
V. Describe the genetic significance of crossing over and independent assortment in meiosis.
VI. Describe autosomal inheritance.
VII. Describe sex-linked inheritance.
VIII. What is a mutation? Which type of cells would need to mutate for that mutation to be expressed in any offspring?
IX. Describe the chromosome abnormalities that result in the following genetic disorders.
Learning Objectives and Guiding Questions
At the end of this unit, you should be able to complete all the following tasks, including answering the guiding questions associated with each task.
I. Distinguish between significant related genetic terms.
- Distinguish between gene and allele
- Distinguish between character and trait
- Distinguish between genotype and phenotype
- Distinguish between chromatin, chromosome and chromatid
- Distinguish between haploid and diploid
II. Describe the karyotype of a normal human female and the karyotype of a normal human male.
III. Describe the processes of mitosis and meiosis.
- Specify where mitosis and meiosis occur in the human body.
- Compare and contrast the functions of mitosis and meiosis.
- Draw diagrams to illustrate the behaviour of chromosomes during mitosis.
- Draw diagrams to illustrate the behaviour of chromosomes during meiosis.
IV. Define the term nondisjunction.
- Describe when it can occur and what the result of it is.
V. Describe the genetic significance of crossing over and independent assortment in meiosis.
VI. Describe autosomal inheritance.
- Distinguish between homozygous and heterozygous genotypes.
- Distinguish between dominant, and recessive alleles
- Specify the genotypes and phenotype of both parents and offspring in the following cases:
-
- Both parents are heterozygous
- One parent is heterozygous, and the other is homozygous for the dominant allele
- One parent is heterozygous, and the other is homozygous for the recessive allele
- Both parents are homozygous for the recessive allele
- Both parents are homozygous for the dominant allele
VII. Describe sex-linked inheritance.
- Specify the genotype and phenotype of both parents and offspring in the following cases:
-
- The mother is heterozygous, and the father has the dominant allele
- The mother is heterozygous, and the father has the recessive allele
- The mother is homozygous for the dominant allele, and the father has the recessive allele
- The mother is homozygous for the dominant allele, and the father has the dominant allele
- The mother is homozygous for the recessive allele, and the father has the recessive allele
- The mother is homozygous for the recessive allele, and the father has the dominant allele
VIII. Define the term mutation and identify the type of cells that would need to mutate for a mutation to be passed on to any offspring?
IX. Describe the chromosome abnormalities that result in the following genetic disorders.
- Klinefelter syndrome
- Turner syndrome
- Down syndrome
Part 1: Cell Growth and Division
While there are a few cells in the body that do not undergo cell division (such as gametes, red blood cells, most neurons, and some muscle cells), most somatic cells divide regularly. A somatic cell is a general term for a body cell, and all human cells, except for the cells that produce eggs and sperm (which are referred to as germ cells), are somatic cells. Somatic cells contain two copies of each of their chromosomes (one copy received from each parent). A homologous pair of chromosomes is the two versions of a single chromosome found in each somatic cell. The human is a diploid organism, having 23 homologous pairs of chromosomes in each of the somatic cells. The condition of having pairs of chromosomes is known as diploidy.
Cells in the body replace themselves over the lifetime of a person. For example, the cells lining the gastrointestinal tract must be frequently replaced when constantly “worn off” by the movement of food through the gut. But what triggers a cell to divide, and how does it prepare for and complete cell division? The cell cycle is the sequence of events in the life of the cell from the moment it is created at the end of a previous cycle of cell division until it then divides itself, generating two new cells.
The Cell Cycle
One “turn” or cycle of the cell cycle consists of two general phases: interphase, followed by cell division (mitosis and cytokinesis). Interphase is the period of the cell cycle during which the cell is not dividing. The majority of cells are in interphase most of the time. Mitosis is the division of genetic material, during which the cell nucleus breaks down and two new, fully functional, nuclei are formed. Cytokinesis divides the cytoplasm into two distinctive cells.
Interphase: A cell grows and carries out all normal metabolic functions and processes in a period called G1 (Figure 1). G1 phase (gap 1 phase) is the first gap, or growth phase in the cell cycle. For cells that will divide again, G1 is followed by replication of the DNA, during the S phase. The S phase (synthesis phase) is period during which a cell replicates its DNA.
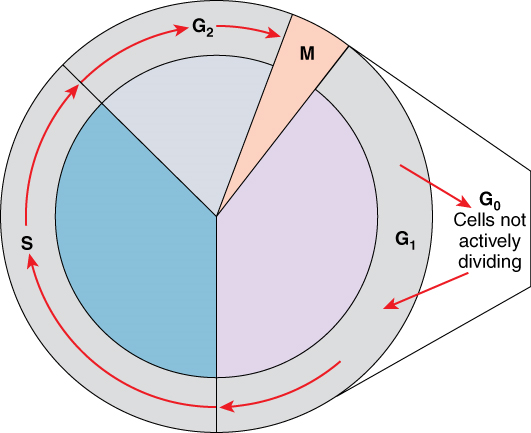
After the synthesis phase, the cell proceeds through the G2 phase. The G2 phase is a second gap phase, during which the cell continues to grow and makes the necessary preparations for mitosis. Between G1, S, and G2 phases, cells will vary the most in their duration of the G1 phase. It is here that a cell might spend a couple of hours, or many days. The S phase typically lasts between 8-10 hours and the G2 phase approximately 5 hours. In contrast to these phases, the G0 phase is a resting phase of the cell cycle. Cells that have temporarily stopped dividing and are resting (a common condition) and cells that have permanently ceased dividing (like nerve cells) are said to be in G0.
The Structure of Chromosomes
Billions of cells in the human body divide every day. During the synthesis phase (S, for DNA synthesis) of interphase, the amount of DNA within the cell precisely doubles. Therefore, after DNA replication but before cell division, each cell actually contains two copies of each chromosome. Each copy of the chromosome is referred to as a sister chromatid and is physically bound to the other copy. The centromere is the structure that attaches one sister chromatid to another. Because a human cell has 46 chromosomes, during this phase, there are 92 chromatids (46 × 2) in the cell. Make sure not to confuse the concept of a pair of chromatids (one chromosome and its exact copy attached during mitosis) and a homologous pair of chromosomes (two paired chromosomes which were inherited separately, one from each parent) (Figure 2).
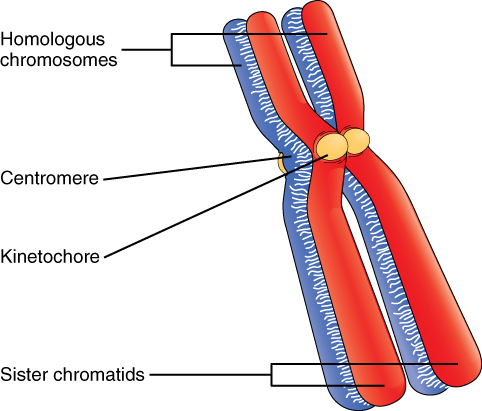
Mitosis and Cytokinesis
The mitotic phase of the cell typically takes between 1 and 2 hours. During this phase, a cell undergoes two major processes. First, it completes mitosis, during which the contents of the nucleus are equitably pulled apart and distributed between its two halves. Cytokinesis then occurs, dividing the cytoplasm and cell body into two new cells. Mitosis is divided into four major stages that take place after interphase (Figure 3) and in the following order: prophase, metaphase, anaphase, and telophase. The process is then followed by cytokinesis.
Prophase is the first phase of mitosis, during which the loosely packed chromatin coils and condenses into visible chromosomes. During prophase, each chromosome becomes visible forming the familiar X-shape of sister chromatids. The nucleolus disappears early during this phase, and the nuclear envelope also disintegrates. A major occurrence during prophase concerns a very important structure that contains the origin site for microtubule growth. Recall the cellular structures called centrioles that serve as origin points from which microtubules extend. These tiny structures also play a very important role during mitosis. A centrosome is a pair of centrioles together. The cell contains two centrosomes side-by-side, which begin to move apart during prophase. As the centrosomes migrate to two different sides of the cell, microtubules begin to extend from each like long fingers from two hands extending toward each other. The mitotic spindle is the structure composed of the centrosomes and their emerging microtubules.
Near the end of prophase there is an invasion of the nuclear area by microtubules from the mitotic spindle. The nuclear membrane has disintegrated, and the microtubules attach themselves to the centromeres that adjoin pairs of sister chromatids. The kinetochore is a protein structure on the centromere that is the point of attachment between the mitotic spindle and the sister chromatids. This stage is referred to as late prophase or prometaphase to indicate the transition between prophase and metaphase.
Metaphase is the second stage of mitosis. During this stage, the sister chromatids, with their attached microtubules, line up along an imaginary linear plane in the middle of the cell, called the metaphase plate. The microtubules are now poised to pull apart the sister chromatids and bring one from each pair to each side of the cell.
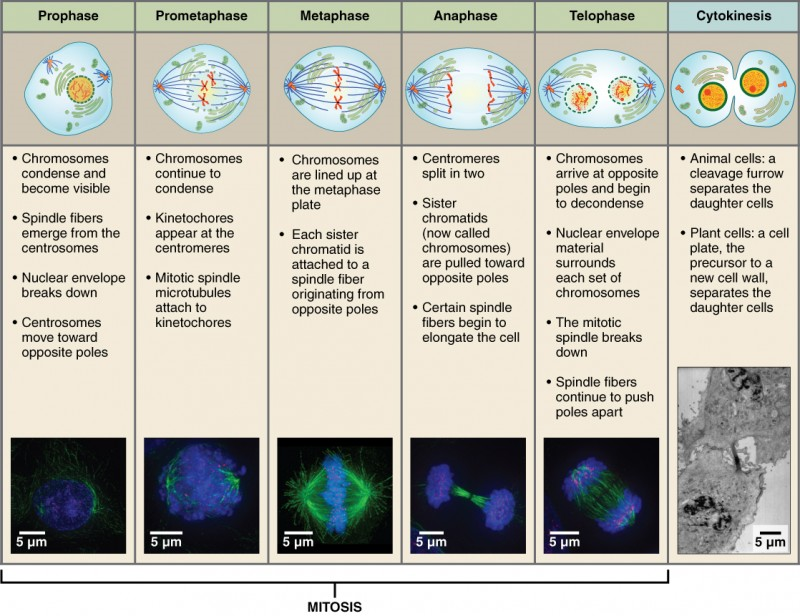
Anaphase is the third stage of mitosis. Anaphase takes place over a few minutes, when the pairs of sister chromatids are separated from one another, forming individual chromosomes once again. These chromosomes are pulled to opposite ends of the cell by their kinetochores, as the microtubules shorten. Each end of the cell receives one partner from each pair of sister chromatids, ensuring that the two new daughter cells will contain identical genetic material.
Telophase is the final stage of mitosis. Telophase is characterized by the formation of two new daughter nuclei at either end of the dividing cell. These newly formed nuclei surround the genetic material, which uncoils such that the chromosomes return to loosely packed chromatin. Nucleoli also reappear within the new nuclei, and the mitotic spindle breaks apart, each new cell receiving its own complement of DNA, organelles, membranes, and centrioles. At this point, the cell is already beginning to split in half as cytokinesis begins.
The cleavage furrow is a contractile band made up of microfilaments that forms around the midline of the cell during cytokinesis. (Recall that microfilaments consist of actin.) This contractile band squeezes the two cells apart until they finally separate. Two new cells are now formed. One of these cells (the “stem cell”) enters its own cell cycle; able to grow and divide again at some future time. The other cell transforms into the functional cell of the tissue, typically replacing an “old” cell there.
Meiosis
Meiosis, unlike mitosis, is not part of the cell cycle of most cells, but only of the germ cells. The daughter cells generated by meiosis are four haploid cells that are each genetically different to the parent cell.
Meiosis is divided into two major stages, meiosis I and meiosis II, that is each further divided into four main stages that are similar to those of mitosis: prophase, metaphase, anaphase, and telophase (Figure 4).
Prophase I is the first phase of meiosis, during which the loosely packed chromatin coils and condenses into visible chromosomes, in a manner similar to prophase of mitosis. In prophase I, however, homologous chromosomes – chromosomes that contain the same genes – pair together and exchange genetic information with each other. Although pairs of chromosomes contain the same genes, they may contain different variants of those genes known as alleles. This process, known as crossing over, can occur at many points along a chromosome’s length, contributing to genetic variation and resulting in chromosomes that may contain chromatids that are no longer identical to each other.
Metaphase I is the second stage of meiosis. During this stage, the pairs of homologous chromosomes line up along a linear plane in the middle of the cell. Similar to mitosis, the central location where the chromosomes line up is called a metaphase plate. However, unlike mitosis the chromosomes are lined up in pairs. These pairs are arranged in somewhat random orientations relative to each other, in that although they are all lined up at the metaphase plate, the maternal and paternal chromosomes are not necessarily all on the same side of the plate. This lack of regard to the orientation of other chromosomes results in the independent assortment of maternal and paternal genetic information into separate daughter cells.
Anaphase I is the third stage of meiosis. Microtubules pull entire chromosomes to opposite sides of the cell, while leaving the individual chromatids paired. This results in half as many chromosomes being delivered to either side of the cell as were found in the original parent cell.
Telophase I is the final stage of meiosis I; much like telophase of mitosis, telophase I results in the formation of two new daughter nuclei at either end of the dividing cell, surrounding the genetic material. However, in this case each daughter cell has only half of the number of chromosomes of the parent cell, and may have a different complement of alleles than the parent cell. Each chromosome at this stage still consists of two chromatids that then need to be separated.
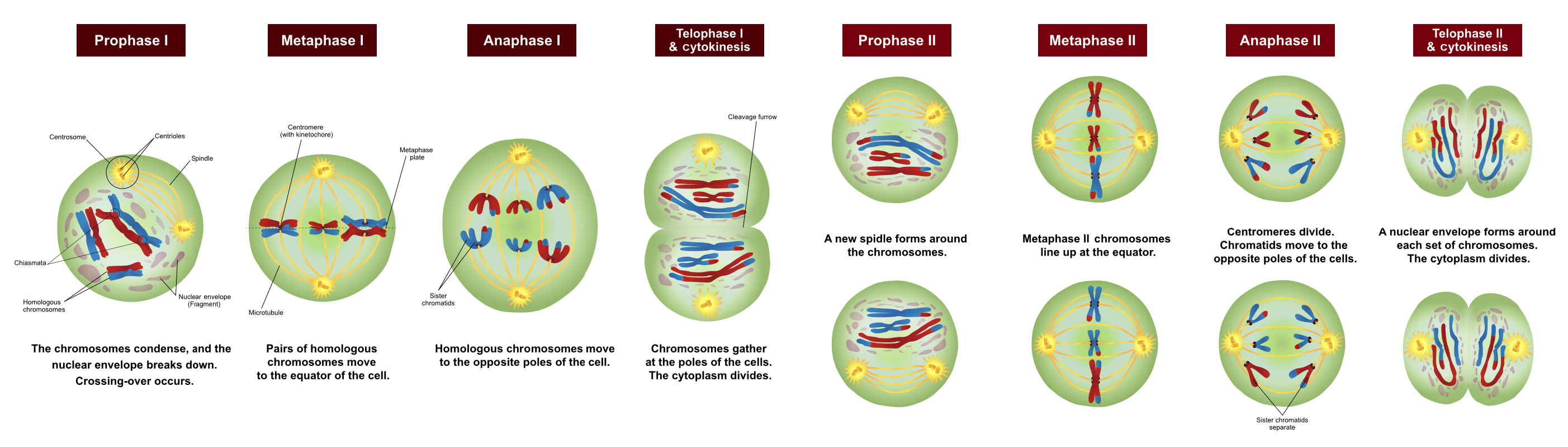
Each of the two cells resulting from meiosis I will therefore need to go through a second round of division known as meiosis II. The behaviour of chromosomes in meiosis II is remarkably similar to that of chromosomes during mitosis. However, cells that enter meiosis II have half as many chromosomes as a cell entering mitosis.
Prophase II is the fifth phase of meiosis and the first phase of meiosis II. Again, chromatin is condensed into visible chromosomes and spindle fibers form.
Metaphase II is the second stage of meiosis II. During this stage, the chromosomes line up along the metaphase plate. As in mitosis, the chromosomes are unpaired and simply line up along the central region of the cell.
Anaphase II is the third stage of meiosis II. Microtubules pull the two chromatids of each chromosome to opposite ends of the cell.
Telophase II is the final stage of meiosis. Since the original parent cell produced two cells that then went on to divide a second time, there are now a total of four daughter cells, each having half the genetic material of the original parental cell. Due to crossing-over between chromosomes and the independent assortment of chromosomes that occurred during meiosis, the four resulting daughter cells are likely to be genetically different from each other.
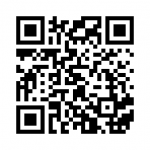
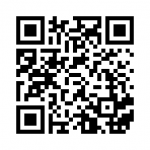
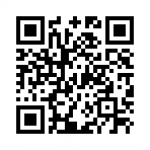
Part 2: Patterns of Inheritance
We have discussed the events that lead to the development of a newborn. But what makes each newborn unique? The answer lies, of course, in the DNA in the sperm and oocyte that combined to produce that first diploid cell, the human zygote.
From Genotype to Phenotype
Each human body cell has a full complement of DNA stored in 23 pairs of chromosomes that can be organized in a systematic way in an arrangement called a karyotype (Figure 5). Among these is one pair of chromosomes, called the sex chromosomes, that determines the sex of the individual (XX in females, XY in males). The remaining 22 chromosome pairs are called autosomal chromosomes. Each of these chromosomes carries hundreds or even thousands of genes, each of which codes for the assembly of a particular protein—that is, genes are “expressed” as proteins. An individual’s complete genetic makeup is referred to as his or her genotype. The characteristics that the genes express, whether they are physical, behavioural, or biochemical, are a person’s phenotype.
You inherit one chromosome in each pair—a full complement of 23—from each parent. This occurs when the sperm and oocyte combine at the moment of your conception. Homologous chromosomes—those that make up a complementary pair—have genes for the same characteristics in the same location on the chromosome. Because one copy of a gene, an allele, is inherited from each parent, the alleles in these complementary pairs may vary. Take for example an allele that encodes for dimples. A child may inherit the allele encoding for dimples on the chromosome from the father and the allele that encodes for smooth skin (no dimples) on the chromosome from the mother.
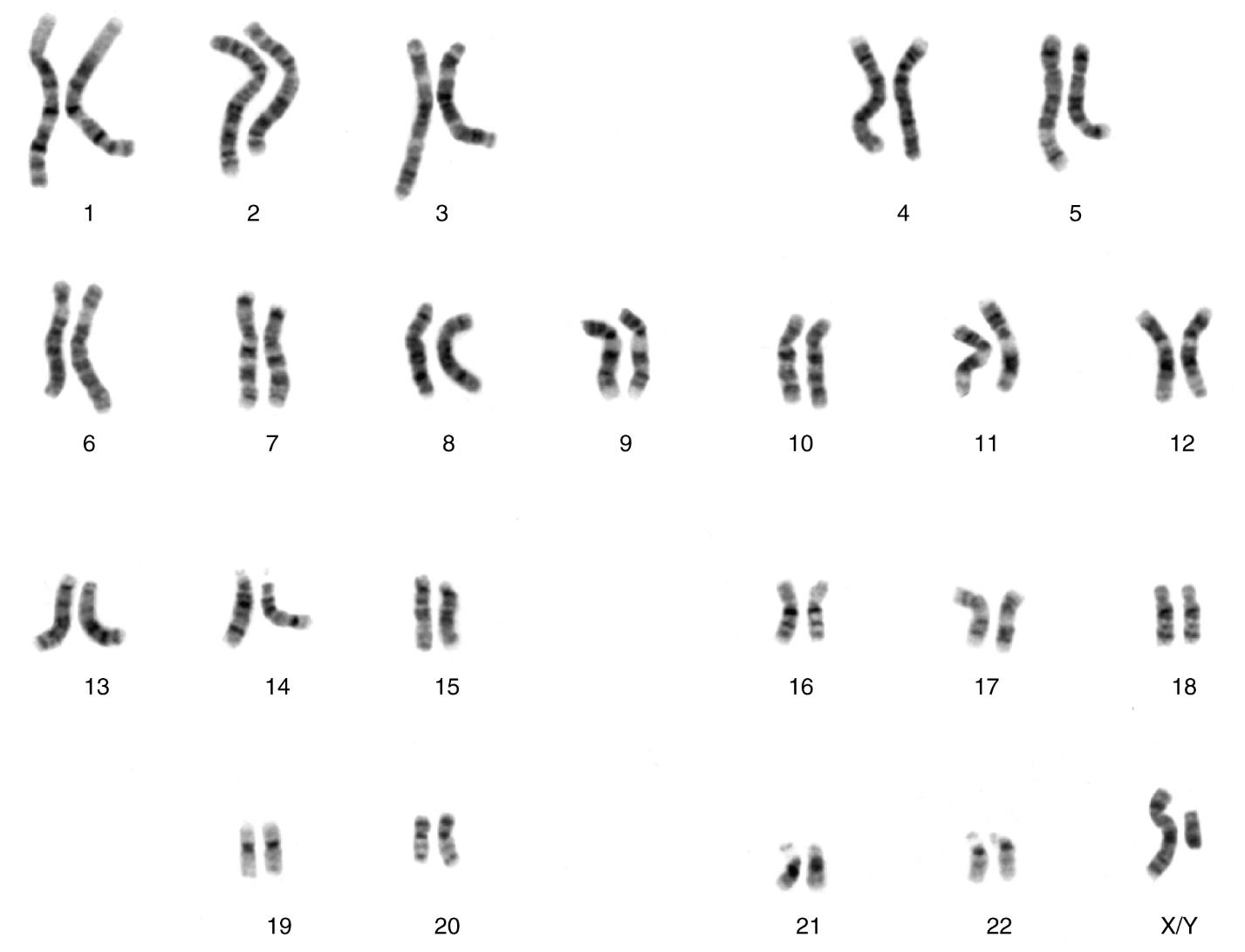
Although a person can have two identical alleles for a single gene (a homozygous state), it is also possible for a person to have two different alleles (a heterozygous state). The two alleles can interact in several different ways. The expression of an allele can be dominant, for which the activity of this gene will mask the expression of a nondominant, or recessive, allele. Sometimes dominance is complete; at other times, it is incomplete. In some cases, both alleles are expressed at the same time in a form of expression known as codominance.
In the simplest scenario, a single pair of genes will determine a single heritable characteristic. However, it is quite common for multiple genes to interact to confer a feature. For instance, eight or more genes—each with their own alleles—determine eye color in humans. Moreover, although any one person can only have two alleles corresponding to a given gene, more than two alleles commonly exist in a population. This phenomenon is called multiple alleles. For example, there are three different alleles that encode ABO blood type; these are designated IA, IB, and i.
Over 100 years of theoretical and experimental genetics studies, and the more recent sequencing and annotation of the human genome, have helped scientists to develop a better understanding of how an individual’s genotype is expressed as their phenotype. This body of knowledge can help scientists and medical professionals to predict, or at least estimate, some of the features that an offspring will inherit by examining the genotypes or phenotypes of the parents. One important application of this knowledge is to identify an individual’s risk for certain heritable genetic disorders. However, most diseases have a multigenic pattern of inheritance and can also be affected by the environment, so examining the genotypes or phenotypes of a person’s parents will provide only limited information about the risk of inheriting a disease. Only for a handful of single-gene disorders can genetic testing allow clinicians to calculate the probability with which a child born to the two parents tested may inherit a specific disease.
Mendel’s Theory of Inheritance
Our contemporary understanding of genetics rests on the work of a nineteenth-century monk. Working in the mid-1800s, long before anyone knew about genes or chromosomes, Gregor Mendel discovered that garden peas transmit their physical characteristics to subsequent generations in a discrete and predictable fashion. When he mated, or crossed, two true-breeding (pure-breeding) pea plants that differed by a certain characteristic, the first-generation offspring all looked like one of the parents. For instance, when he crossed tall and dwarf true-breeding pea plants, all of the offspring were tall. Mendel called tallness dominant because it was expressed in offspring when it was present in a purebred parent. He called dwarfism recessive because it was masked in the offspring if one of the purebred parents possessed the dominant characteristic. Note that tallness and dwarfism are variations on the characteristic of height. Mendel called such a variation a trait. We now know that these traits are the expression of different alleles of the gene encoding height.
Mendel performed thousands of crosses in pea plants with differing traits for a variety of characteristics. And he repeatedly came up with the same results—among the traits he studied, one was always dominant, and the other was always recessive. (Remember, however, that this dominant–recessive relationship between alleles is not always the case; some alleles are codominant, and sometimes dominance is incomplete.)
Using his understanding of dominant and recessive traits, Mendel tested whether a recessive trait could be lost altogether in a pea lineage or whether it would resurface in a later generation. By crossing the second-generation offspring of purebred parents with each other, he showed that the latter was true: recessive traits reappeared in third-generation plants in a ratio of 3:1 (three offspring having the dominant trait and one having the recessive trait). Mendel then proposed that characteristics such as height were determined by heritable “factors” that were transmitted, one from each parent, and inherited in pairs by offspring.
In the language of genetics, Mendel’s theory applied to humans says that if an individual receives two dominant alleles, one from each parent, the individual’s phenotype will express the dominant trait. If an individual receives two recessive alleles, then the recessive trait will be expressed in the phenotype. Individuals who have two identical alleles for a given gene, whether dominant or recessive, are said to be homozygous for that gene (homo- = “same”). Conversely, an individual who has one dominant allele and one recessive allele is said to be heterozygous for that gene (hetero- = “different” or “other”). In this case, the dominant trait will be expressed, and the individual will be phenotypically identical to an individual who possesses two dominant alleles for the trait.
It is common practice in genetics to use capital and lowercase letters to represent dominant and recessive alleles. Using Mendel’s pea plants as an example, if a tall pea plant is homozygous, it will possess two tall alleles (TT). A dwarf pea plant must be homozygous because its dwarfism can only be expressed when two recessive alleles are present (tt). A heterozygous pea plant (Tt) would be tall and phenotypically indistinguishable from a tall homozygous pea plant because of the dominant tall allele. Mendel deduced that a 3:1 ratio of dominant to recessive would be produced by the random segregation of heritable factors (genes) when crossing two heterozygous pea plants. In other words, for any given gene, parents are equally likely to pass down either one of their alleles to their offspring in a haploid gamete, and the result will be expressed in a dominant–recessive pattern if both parents are heterozygous for the trait.
Because of the random segregation of gametes, the laws of chance and probability come into play when predicting the likelihood of a given phenotype. Consider a cross between an individual with two dominant alleles for a trait (AA) and an individual with two recessive alleles for the same trait (aa). All of the parental gametes from the dominant individual would be A, and all of the parental gametes from the recessive individual would be a (Figure 6). All of the offspring of that second generation, inheriting one allele from each parent, would have the genotype Aa, and the probability of expressing the phenotype of the dominant allele would be 4 out of 4, or 100 percent.
This seems simple enough, but the inheritance pattern gets interesting when the second-generation Aa individuals are crossed. In this generation, 50 percent of each parent’s gametes are A and the other 50 percent are a. By Mendel’s principle of random segregation, the possible combinations of gametes that the offspring can receive are AA, Aa, aA (which is the same as Aa), and aa. Because segregation and fertilization are random, each offspring has a 25 percent chance of receiving any of these combinations. Therefore, if an Aa × Aa cross were performed 1000 times, approximately 250 (25 percent) of the offspring would be AA; 500 (50 percent) would be Aa (that is, Aa plus aA); and 250 (25 percent) would be aa. The genotypic ratio for this inheritance pattern is 1:2:1. However, we have already established that AA and Aa (and aA) individuals all express the dominant trait (i.e., share the same phenotype), and can therefore be combined into one group. The result is Mendel’s third-generation phenotypic ratio of 3:1.
Mendel’s observation of pea plants also included many crosses that involved multiple traits, which prompted him to formulate the principle of independent assortment. The law states that the members of one pair of genes (alleles) from a parent will sort independently from other pairs of genes during the formation of gametes. Applied to pea plants, that means that the alleles associated with the different traits of the plant, such as color, height, or seed type, will sort independently of one another. This holds true except when two alleles happen to be located close to one other on the same chromosome. Independent assortment provides for a great degree of diversity in offspring.
Mendelian genetics represent the fundamentals of inheritance, but there are two important qualifiers to consider when applying Mendel’s findings to inheritance studies in humans. First, as we’ve already noted, not all genes are inherited in a dominant–recessive pattern. Although all diploid individuals have two alleles for every gene, allele pairs may interact to create several types of inheritance patterns, including incomplete dominance and codominance.
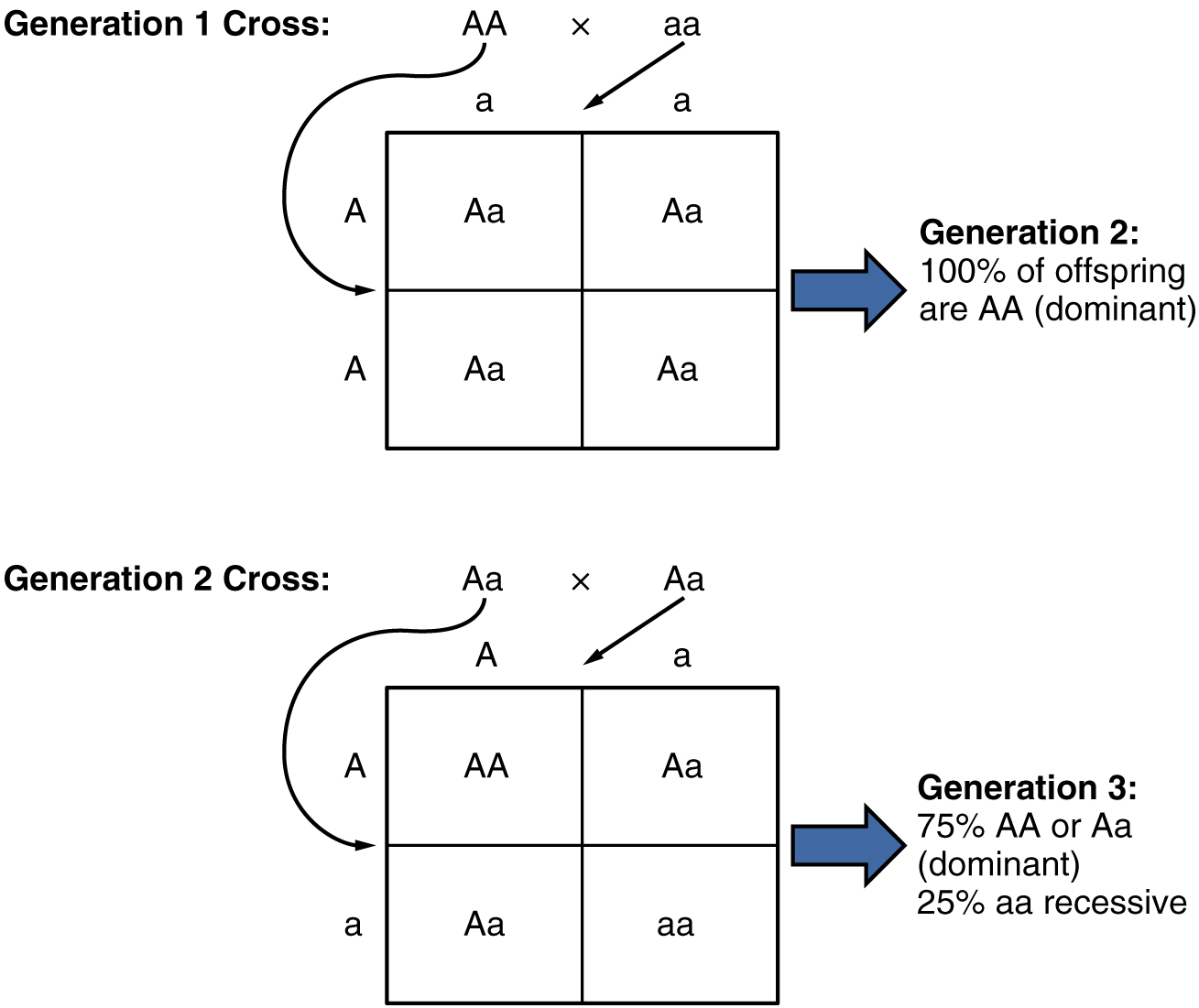
Secondly, Mendel performed his studies using thousands of pea plants. He was able to identify a 3:1 phenotypic ratio in second-generation offspring because his large sample size overcame the influence of variability resulting from chance. In contrast, no human couple has ever had thousands of children. If we know that a man and woman are both heterozygous for a recessive genetic disorder, we would predict that one in every four of their children would be affected by the disease. In real life, however, the influence of chance could change that ratio significantly. For example, if a man and a woman are both heterozygous for cystic fibrosis, a recessive genetic disorder that is expressed only when the individual has two defective alleles, we would expect one in four of their children to have cystic fibrosis. However, it is entirely possible for them to have seven children, none of whom is affected, or for them to have two children, both of whom are affected. For each individual child, the presence or absence of a single gene disorder depends on which alleles that child inherits from his or her parents.
Autosomal Dominant Inheritance
In the case of cystic fibrosis, the disorder is recessive to the normal phenotype. However, a genetic abnormality may be dominant to the normal phenotype. When the dominant allele is located on one of the 22 pairs of autosomes (non-sex chromosomes), we refer to its inheritance pattern as autosomal dominant. An example of an autosomal dominant disorder is neurofibromatosis type I, a disease that induces tumor formation within the nervous system that leads to skin and skeletal deformities. Consider a couple in which one parent is heterozygous for this disorder (and who therefore has neurofibromatosis), Nn, and one parent is homozygous for the normal gene, nn. The heterozygous parent would have a 50 percent chance of passing the dominant allele for this disorder to his or her offspring, and the homozygous parent would always pass the normal allele. Therefore, four possible offspring genotypes are equally likely to occur: Nn, Nn, nn, and nn. That is, every child of this couple would have a 50 percent chance of inheriting neurofibromatosis. This inheritance pattern is shown in Figure 7, in a form called a Punnett square, named after its creator, the British geneticist Reginald Punnett.
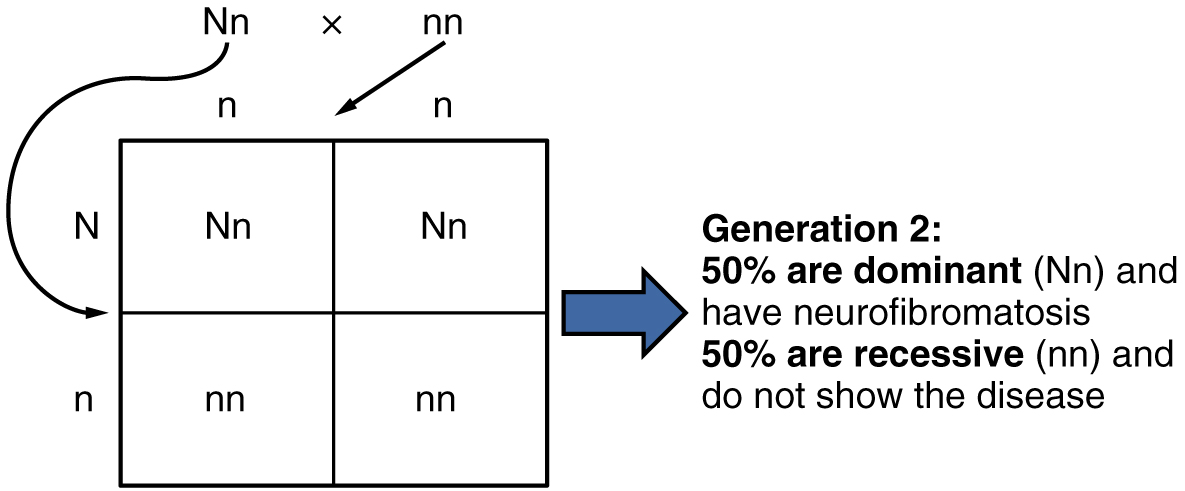
Other genetic diseases that are inherited in this pattern are achondroplastic dwarfism, Marfan syndrome, and Huntington’s disease. Because autosomal dominant disorders are expressed by the presence of just one gene, an individual with the disorder will know that he or she has at least one faulty gene. The expression of the disease may manifest later in life, after the childbearing years, which is the case in Huntington’s disease (discussed in more detail later in this section).
Autosomal Recessive Inheritance
When a genetic disorder is inherited in an autosomal recessive pattern, the disorder corresponds to the recessive phenotype. Heterozygous individuals will not display symptoms of this disorder, because their unaffected gene will compensate. Such an individual is called a carrier. Carriers for an autosomal recessive disorder may never know their genotype unless they have a child with the disorder.
An example of an autosomal recessive disorder is cystic fibrosis (CF). CF is characterized by the chronic accumulation of a thick, tenacious mucus in the lungs and digestive tract. Decades ago, children with CF rarely lived to adulthood. With advances in medical technology, the average lifespan in developed countries has increased into middle adulthood. CF is a relatively common disorder that occurs in approximately 1 in 2000 Caucasians. A child born to two CF carriers would have a 25 percent chance of inheriting the disease. This is the same 3:1 dominant: recessive ratio that Mendel observed in his pea plants would apply here. The pattern is shown in Figure 8, using a diagram that tracks the likely incidence of an autosomal recessive disorder on the basis of parental genotypes.
On the other hand, a child born to a CF carrier and someone with two unaffected alleles would have a 0 percent probability of inheriting CF, but would have a 50 percent chance of being a carrier. Other examples of autosome recessive genetic illnesses include the blood disorder sickle-cell anemia, the fatal neurological disorder Tay–Sachs disease, and the metabolic disorder phenylketonuria.
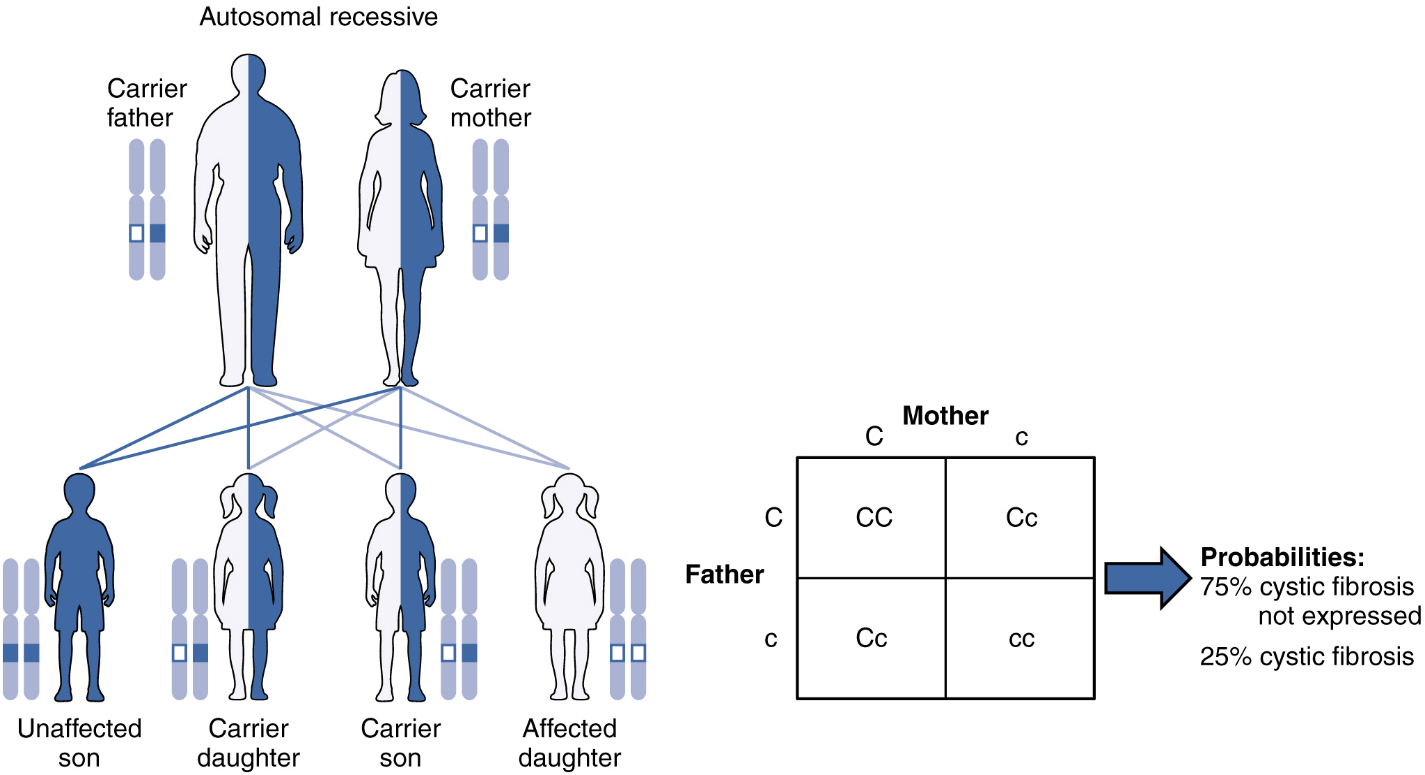
X-linked Dominant or Recessive Inheritance
An X-linked transmission pattern involves genes located on the X chromosome of the 23rd pair (Figure 9). Recall that a male typically has one X and one Y chromosome. When a father transmits a Y chromosome, the child is genetically male, and when he transmits an X chromosome, the child is genetically female. A mother can transmit only an X chromosome, as both her sex chromosomes are X chromosomes.
For genes on either sex chromosome, when examining inheritance it is important to keep track of which chromosome they are on as well as the allele present. When an abnormal allele for a gene that occurs on the X chromosome is dominant over the normal allele, the pattern is described as X-linked dominant. Such an allele would be symbolized using a capital letter superscript on a capital X, e.g.: XA. Thus an otherwise normal individual carrying a dominant allele of the X-linked gene “A” could have any of the following genotypes: XAXA (female, homozygous dominant, abnormal phenotype), XAXa (female, heterozygous, abnormal phenotype), XAY (male, abnormal phenotype). Note that for any X-linked gene, males are expected to only have a single allele because they normally only have a single X chromosome, whereas females will be expected to have two alleles – that may be the same or different – because they normally have two X chromosomes.
An example of an X-linked dominant trait is vitamin D–resistant rickets: an affected father would pass the disease allele to all of his daughters, but none of his sons, because he donates only the Y chromosome to his sons (Figure 9a). If it is the mother who is affected, all of her children—male or female—would have a 50 percent chance of inheriting the disorder because she can only pass an X chromosome on to her children (Figure 9b). For an affected female, the inheritance pattern would be identical to that of an autosomal dominant inheritance pattern in which one parent is heterozygous and the other is homozygous for the normal gene.
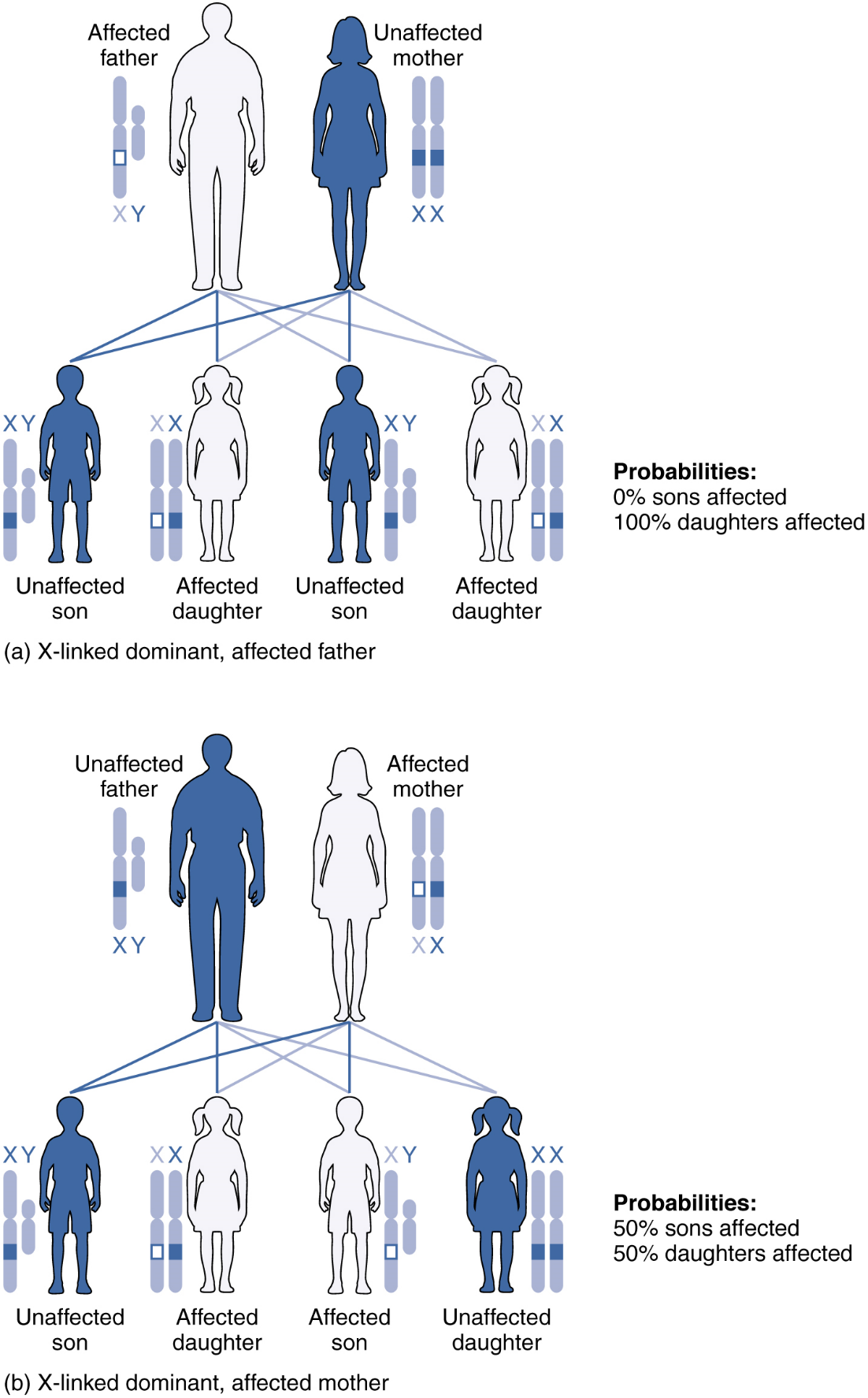
X-linked recessive inheritance is much more common because females can be carriers of the disease yet still have a normal phenotype. This inheritance pattern occurs when an abnormal allele for a gene that occurs on the X chromosome is recessive to the normal allele. Such an allele would be symbolized using a lower-case letter superscript on a capital X, e.g.: Xb. Thus an otherwise normal individual carrying the abnormal allele of the X-linked gene “B” could have any of the following genotypes: XBXb (female, heterozygous, normal phenotype), XbXb (female, homozygous recessive, abnormal phenotype), XbY (male, abnormal phenotype). Again, for an X-linked gene males are expected to have only a single allele (on their one X chromosome), whereas females are expected to have two alleles that may be the same or different (one on each of their two X chromosomes).
Diseases transmitted by X-linked recessive inheritance include color blindness, the blood-clotting disorder hemophilia, and some forms of muscular dystrophy. For an example of X-linked recessive inheritance, consider parents in which the mother is an unaffected carrier and the father is normal. None of the daughters would have the disease because they receive a normal gene from their father. However, they have a 50 percent chance of receiving the disease gene from their mother and becoming a carrier. In contrast, 50 percent of the sons would be affected (Figure 10).
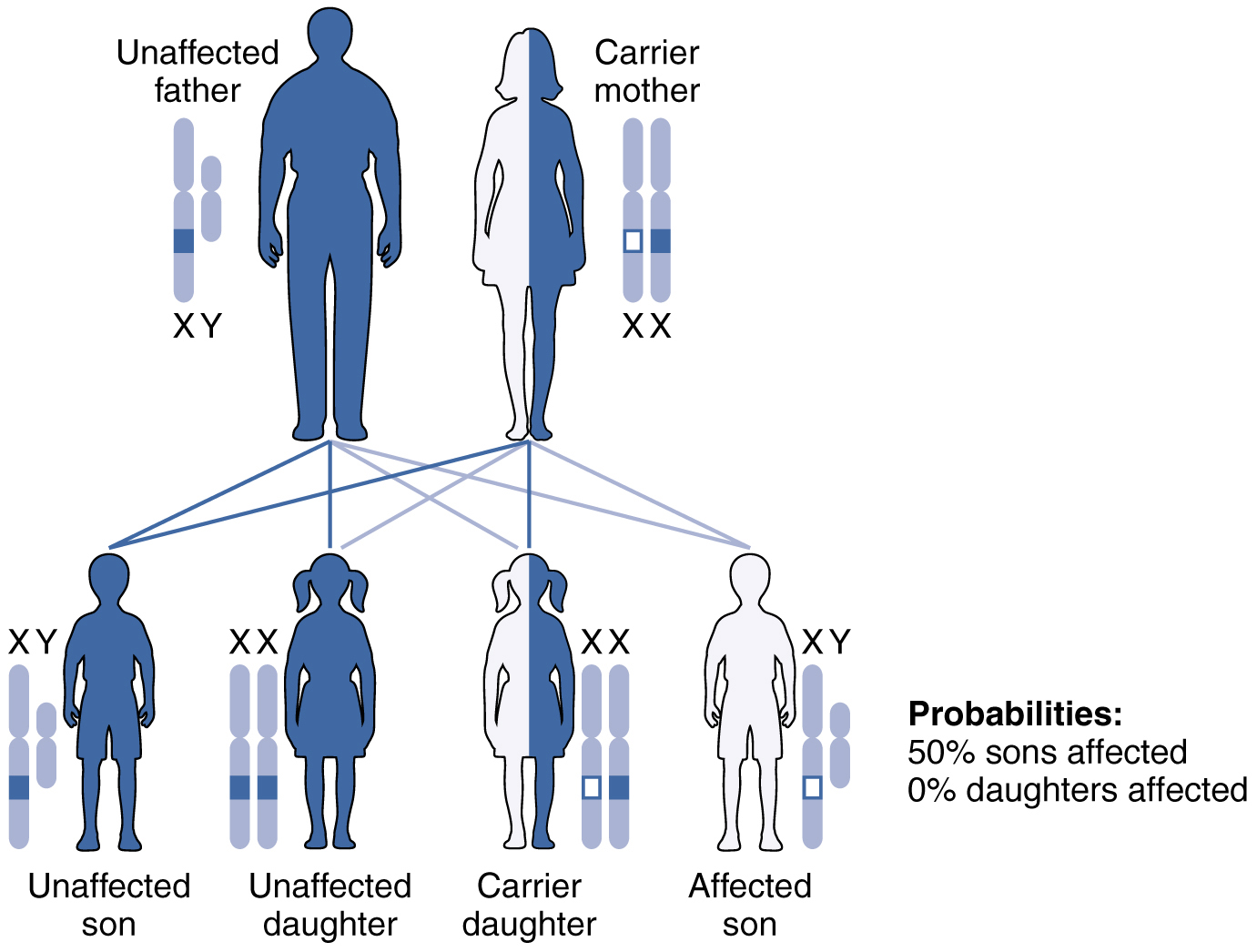
With X-linked recessive diseases, males either have the disease or are genotypically normal—they cannot be carriers. Females, however, can be genotypically normal, a carrier who is phenotypically normal, or affected with the disease. A daughter can inherit the gene for an X-linked recessive illness when her mother is a carrier or affected, or her father is affected. The daughter will be affected by the disease only if she inherits an X-linked recessive gene from both parents. As you can imagine, X-linked recessive disorders affect many more males than females. For example, color blindness affects at least 1 in 20 males, but only about 1 in 400 females.
Certain combinations of alleles can be lethal, meaning they prevent the individual from developing in utero, or cause a shortened life span. In recessive lethal inheritance patterns, a child who is born to two heterozygous (carrier) parents and who inherited the faulty allele from both would not survive. An example of this is Tay–Sachs, a fatal disorder of the nervous system. In this disorder, parents with one copy of the allele for the disorder are carriers. If they both transmit their abnormal allele, their offspring will develop the disease and will die in childhood, usually before age 5.
Dominant lethal inheritance patterns are much rarer because neither heterozygotes nor homozygotes survive. Of course, dominant lethal alleles that arise naturally through mutation and cause miscarriages or stillbirths are never transmitted to subsequent generations. However, some dominant lethal alleles, such as the allele for Huntington’s disease, cause a shortened life span but may not be identified until after the person reaches reproductive age and has children. Huntington’s disease causes irreversible nerve cell degeneration and death in 100 percent of affected individuals, but it may not be expressed until the individual reaches middle age. In this way, dominant lethal alleles can be maintained in the human population. Individuals with a family history of Huntington’s disease are typically offered genetic counseling, which can help them decide whether or not they wish to be tested for the faulty gene.
Mutations
A mutation is a change in the sequence of DNA nucleotides that may or may not affect a person’s phenotype. Mutations can arise spontaneously from errors during DNA replication, or they can result from environmental insults such as radiation, certain viruses, or exposure to tobacco smoke or other toxic chemicals. Because genes encode for the assembly of proteins, a mutation in the nucleotide sequence of a gene can change amino acid sequence and, consequently, a protein’s structure and function. Spontaneous mutations occurring during meiosis are thought to account for many spontaneous abortions (miscarriages).
Chromosomal Disorders
Sometimes a genetic disease is not caused by a mutation in a gene, but by the presence of an incorrect number of chromosomes. Nondisjunction is the term used in genetics to describe how chromosomes fail to disjoin and move to opposite poles during either Meiosis I or Meiosis II. For example, Down syndrome is caused by having three copies of chromosome 21. This is known as trisomy 21. The most common cause of trisomy 21 is chromosomal nondisjunction during meiosis in the mother. The frequency of nondisjunction events appears to increase with age, so the frequency of bearing a child with Down syndrome increases in women over 36.
Whereas Down syndrome is caused by having three copies of a chromosome, Turner syndrome is caused by having just one copy of the X chromosome. This is known as monosomy. The affected child is considered female. Individuals with Turner syndrome are infertile because their sexual organs do not mature.
Having two copies of the X chromosome and one of the Y is also possible and is known as Klinefelter syndrome. The affected child is genetically male, since the Y chromosome is present, and again is infertile. Individuals are normal intellectually, but the incidence of intellectual disability increases as the number of X chromosomes present increases.
Detecting Genetic Disorders
For many genetic diseases, a DNA test can determine whether a person is a carrier. For instance, carrier status for Fragile X, an X-linked disorder associated with mental retardation, or for cystic fibrosis can be determined with a simple blood draw to obtain DNA for testing. A genetic counselor can educate a couple about the implications of such a test and help them decide whether to undergo testing. For chromosomal disorders, the available testing options include a blood test, amniocentesis (in which amniotic fluid is tested), and chorionic villus sampling (in which tissue from the placenta is tested). Each of these has advantages and drawbacks. A genetic counselor can also help a couple cope with the news that either one or both partners is a carrier of a genetic illness, or that their unborn child has been diagnosed with a chromosomal disorder or other birth defect.
A body cell, excluding germ cells. Normally diploid, each cell containing a complete set of genes.
Cell that gives rise to a gamete.
Having two copies of genetic material.
The life cycle of a cell, including interphase and mitotic phases.
Division of genetic material, during which the cell nucleus breaks down and two new, fully functional, nuclei are formed. Usually immediately followed by cytokinesis (cell division).
Division of the cytoplasm to form two separate cells.
Portions of the cell cycle that are not part of mitosis.
A long DNA molecule, combined with proteins that contains a number of genes. The normal chromosome compliment is 23 pairs of homologous chromosomes, one each from mother and father.
An identical copy of a chromosome, formed during S phase in preparation for mitosis, attached at the centromere to another sister chromatid.
A structure on a chromosome, where sister chromatids are attached, and where the mitotic spindle attaches.
Pair of similar (but not identical) chromosomes in a diploid cell, containing the same genes but possibly differing in alleles, one inherited from each parent.
(In nervous system) a localized collection of neuron cell bodies that are functionally related; a “center” of neural function (plural= nuclei).
The first phase of mitosis, during which the nucleolus disappears, the nuclear envelope disintegrates, mitotic spindle begins to form, and chromosomes condense.
The second phase of mitosis, during which replicated chromosomes align on the metaphase plate and the mitotic spindle completes.
Third phase of mitosis, during which sister chromatids separate toward opposite poles, and spindle fibres begin to elongate the cell.
Final phase of telophase, during which chromosomes (now separated at opposite poles) decondense and nuclear envelope re-forms.
Cellular structure that organizes microtubules during cell division.
Small, self-replicating organelle that provides the origin for microtubule growth and moves DNA during cell division.
Structure composed of centrosomes and microtubules, responsible for aligning and separating replicated chromosomes and elongating and dividing the cell during mitosis and cytokinesis.
Part of the chromosome's centromere to which the mitotic spindle attaches.
Late prophase of mitosis, during which the mitotic spindle has attached to the kinetochore of each replicated chromosome.
Imaginary medial plane in a mitotic cell, along which replicated chromosomes align during metaphase.
Substance consisting of DNA and associated proteins.
Small region of the nucleus that functions in ribosome synthesis.
Contractile ring that forms around a cell during cytokinesis that pinches the cell into two halves.
Process by which germ cells form four genetically distinct daughter cells (gametes) for sexual reproduction.
One copy of each homologous chromosomes, (half the normal genetic complement), as in gametes.
First phase of meiosis I during which chromatin condenses to form chromosomes, nuclear envelope disintegrates, mitotic spindle forms, and homologous chromosomes pair together and crossing over occurs.
Alternative forms of a gene that occupy a specific locus on a specific gene.
process by which genetic information of homologou, non-sister chromatids, is exchanged during prophase I, thereby increasing genetic variability of gametes.
second phase of meiosis I during which homologous chromosomes align on the metaphase plate.
Third phase of meiosis I, during which homologous chromosomes are separated to opposite poles.
The final phase of meiosis I, during which replicated chromosomes (consisting of paired sister chromatids) form new nuclei at either end of the dividing cell.
The first phase of meiosis II, during which chromatin condenses and the mitotic spindle forms.
The second stage of meiosis II, during which replicated chromosomes (consisting of a pair of sister chromatids) align along the metaphase plate.
The third phase of meiosis II, during which sister chromatids are separated to opposite poles of the cell.
The final phase of meiosis II, resulting in four (from two separate cells each undergoing meiosis II) daughter cells that each genetically unique (from each other and from the parent cell).
Systematic arrangement of images of chromosomes into homologous pairs.
The X and Y chromosomes.
Chromosomes excluding the sex chromosomes.
Portion of a chromosome that codes for the assembly of a particular protein or RNA.
Class of organic compounds that are composed of many amino acids linked together by peptide bonds.
The genetic makeup of an individual. Also referring to the alleles an individual has for a particular gene (e.g. homozygous or heterozygous).
Physical or biochemical manifestation of the genotype; expression of the alleles.
Having two identical alleles for a given gene.
Having two different alleles for a given gene.
(In genetics) describes a trait that is expressed both in homozygous and heterozygous form.
Pattern of inheritance that corresponds to the equal, distinct, and simultaneous expression of two different alleles.
Describes a trait that is only expressed in homozygous form and is masked in heterozygous form.
Variation of an expressed characteristic.
Haploid reproductive cell (egg or sperm in humans) that contributes genetic material to form an offspring.
Predicted ratio of genotypes among offspring.
Predicted ratio of phenotypes among offspring; this may differ from the genotypic ratio for the same cross where certain genotypes (e.g. homozygous dominant and heterozygous) have the same phenotype.
Mendelian principle that states that alleles from one parent will sort independently from those of the other parent. This occurs during metaphase I when maternal and paternal homologous chromosomes align themselves independently to maternal and paternal chromosomes of any other pair.
Pattern of dominant inheritance that corresponds to a gene on one of the 22 autosomal chromosomes.
Grid used to display all possible combinations of alleles transmitted by parents to offspring and predict the mathematical probability of offspring inheriting a given genotype.
Pattern of recessive inheritance that corresponds to a gene on one of the 22 autosomal chromosomes.
(In genetics) heterozygous individual who does not display symptoms of a recessive genetic disorder but can transmit the disorder to his or her offspring.
Pattern of dominant inheritance that corresponds to a gene on the X chromosome of the 23rd pair.
Pattern of recessive inheritance that corresponds to a gene on the X chromosome of the 23rd pair.
Change in the nucleotide sequence of DNA.
A chromosomal disorder in which chromosomes fail to disjoin and move to opposite poles during either Meiosis I or Meiosis II.