Unit 4: Blood Vessels and Circulation
Unit Outline
Part 1: Structure and function of blood vessels
Part 3: Blood flow, blood pressure, and resistance
- Components of arterial blood pressure
- Pulse
- Variables affecting blood flow and blood pressure
- Venous system
Part 4: Hemostatic Regulation of the Vascular System
- Neural regulation
- The cardiovascular centres in the brain
- Baroreceptor reflexes
- Endocrine regulation
- Autoregulation of perfusion
- Pulmonary circulation
- Overview of systemic arteries
- The aorta
- Coronary circulation
- Aortic arch branches
- Thoracic aorta and major branches
- Abdominal aorta and major branches
- Arteries serving the upper and lower limbs
- Overview of systemic veins
- The superior and inferior vena cavae
- Veins draining the lower limbs
Practice Questions
Learning Objectives
At the end of this unit, you should be able to:
I. Describe relationships between the following components of the cardiovascular system and explain their functions: blood, artery, vein, capillary, atria, and ventricles.
II. Compare the structure and function of arteries, veins, and capillaries.
III. Describe what is meant by blood pressure and specify the following: five factors which affect blood pressure, the major mechanisms that control blood pressure, and the average blood pressure of a young adult.
IV. Describe what is felt when a pulse is located, and specify four points where an arterial pulse may be felt.
V. Describe the following components of the cardiovascular system: the main arteries leaving the heart, and those serving the trunk, appendages, and heart; the main veins entering the heart, and those draining the trunk, appendages, and heart.
Learning Objectives and Guiding Questions
At the end of this unit, you should be able to complete all the following tasks, including answering the guiding questions associated with each task.
I. Describe relationships between the following components of the cardiovascular system and explain their functions: blood, artery, vein, capillary, atria, and ventricles.
II. Compare the structure and function of arteries, veins, and capillaries.
- In general, which arteries and veins carry oxygenated and deoxygenated blood?
- Define: artery, arteriole, vein, venule, capillary.
- Compare and contrast the structure of the walls of arteries, veins, and capillaries.
III. Describe what is meant by blood pressure and specify the following: five factors which affect blood pressure, the major mechanisms that control blood pressure, and the average blood pressure of a young adult.
- Define the term “blood pressure”.
- Describe how blood pressure is measured.
- Define cardiac output and describe how each of the following physiological factors affect blood pressure:
- Heart rate
- Contractility (strength of contraction) of the heart
- Blood volume
- Peripheral resistance
- Blood viscosity
- How does maintaining blood pressure contribute to homeostasis.
- Describe how blood pressure is regulated by:
-
- The nervous system
- The endocrine system
- Autoregulation
IV. Describe what is felt when a pulse is located, and specify four points where an arterial pulse may be felt.
- When you manually “take someone’s pulse”, what is causing the pulsing pressure waves you feel?
- List four locations on the human body where a pulse can be taken manually and explain why an arterial pulse can be felt at specific locations rather than just anywhere on the human body.
V. Describe, using examples, how capillaries use simple diffusion, facilitated diffusion and osmosis to exchange material with tissues.
VI. Describe the following components of the cardiovascular system: the main arteries leaving the heart, and those serving the trunk, appendages, and heart; the main veins entering the heart, and those draining the trunk, appendages, and heart.
- Draw a flow chart showing the components of the cardiovascular system. Start with the three main components (heart, blood vessels, and blood), and continue by specifying all the constituent parts of each.
- Compare and contrast (clearly!) the anatomical structure and function of arteries, veins, and blood capillaries.
- Draw a simple diagram of the human cardiovascular system that shows both circuits, indicating the vessels blood is moved through as it is passed to and from the head, arms, organs of the abdomen, and lungs. Your diagram should include:
- The main arteries leaving the heart:
- Pulmonary trunk
- Pulmonary arteries
- Pulmonary veins
- Aorta
- Ascending aorta
- Aortic arch
- The main arteries serving the trunk, appendages and the heart:
- Descending aorta
- Thoracic aorta
- Abdominal aorta
- Brachiocephalic artery
- Left common carotid artery
- Right common carotid artery
- Left subclavian artery
- Right subclavian artery
- Common iliac artery
- Axillary artery
- Femoral artery
- The main veins entering the heart
- Superior vena cava
- Inferior vena cava
- Coronary sinus
- The main veins draining the trunk, appendages and the heart
- Subclavian vein
- Axillary vein
- Brachiocephalic vein
- Femoral vein
- Common iliac vein
- The main arteries leaving the heart:
In this unit, you will learn about the vascular part of the cardiovascular system; that is, the vessels that transport blood throughout the body and provide the physical site where gases, nutrients, and other substances are exchanged with body cells. When vessel functioning is reduced, blood-borne substances do not circulate effectively throughout the body. As a result, tissue injury occurs, metabolism is impaired, and the functions of every bodily system are threatened.
Part 1: Structure and Function of Blood Vessels
Blood is carried through the body via blood vessels. An artery is a blood vessel that carries blood away from the heart, where it branches into ever-smaller vessels. Eventually, the smallest arteries, vessels called arterioles, further branch into tiny capillaries, where nutrients and wastes are exchanged, and then combine with other vessels that exit capillaries to form venules, small blood vessels that carry blood to a vein, a larger blood vessel that returns blood to the heart.
Arteries and veins transport blood in two distinct circuits: the systemic circuit and the pulmonary circuit (Figure 1). Systemic arteries provide blood rich in oxygen to the body’s tissues. The blood returned to the heart through systemic veins has less oxygen, since much of the oxygen carried by the arteries has been delivered to the cells. In contrast, in the pulmonary circuit, arteries carry blood low in oxygen exclusively to the lungs for gas exchange. Pulmonary veins then return freshly oxygenated blood from the lungs to the heart to be pumped back out into systemic circulation. Although arteries and veins differ structurally and functionally, they share certain features.
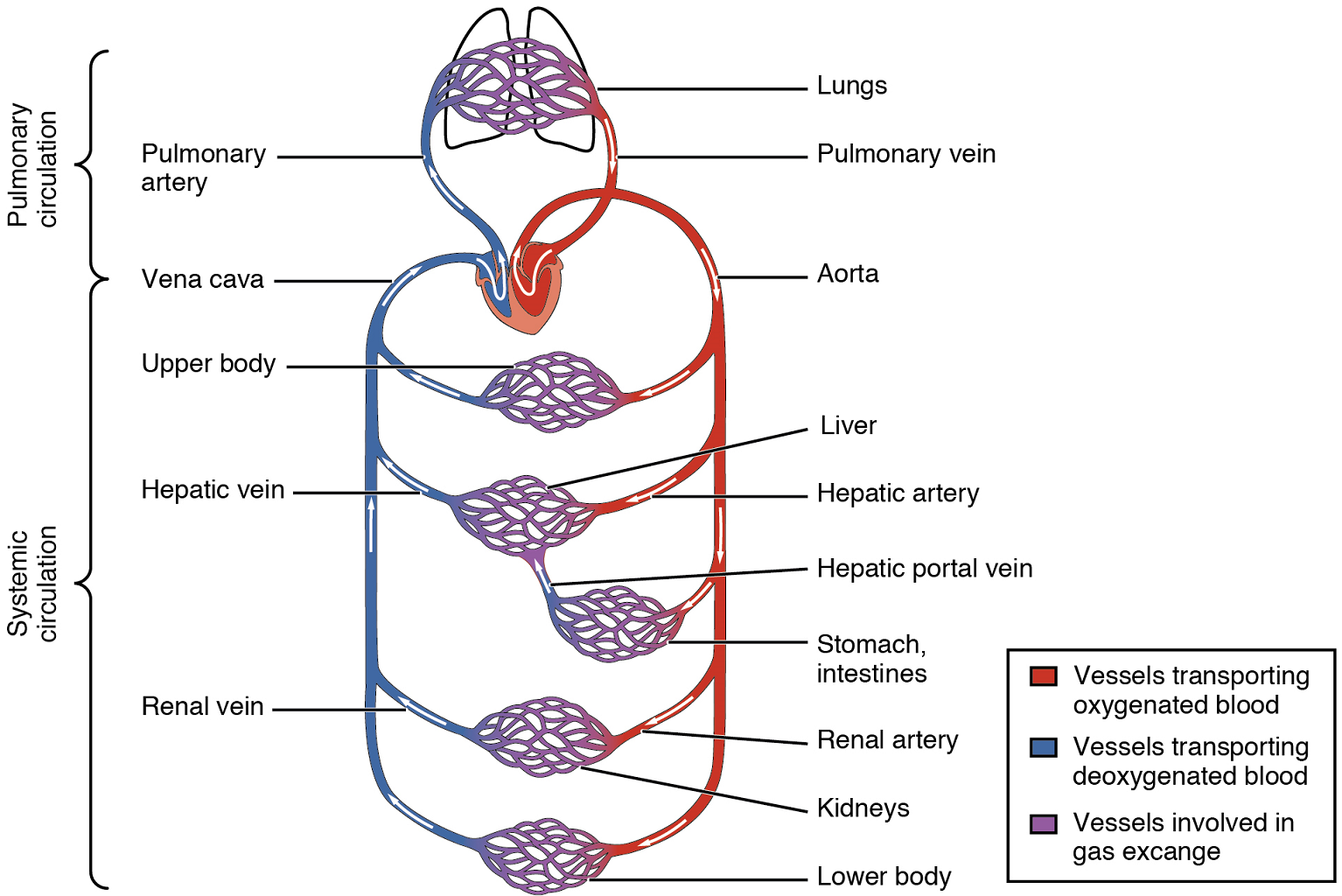
Shared Structures
Different types of blood vessels vary slightly in their structures, but they share the same general features. Arteries and arterioles have thicker walls than veins and venules because they are closer to the heart and receive blood that is surging at a far greater pressure (Figure 2). Each type of vessel has a lumen—a hollow passageway through which blood flows. Arteries have smaller lumens than veins, a characteristic that helps to maintain the pressure of blood moving through the system. Together, their thicker walls and smaller diameters give arterial lumens a more rounded appearance in cross section than the lumens of veins.
By the time blood has passed through capillaries and entered venules, the pressure initially exerted upon it by heart contractions has diminished. In other words, in comparison to arteries, venules and veins withstand a much lower pressure from the blood that flows through them. Their walls are considerably thinner and their lumens are correspondingly larger in diameter, allowing more blood to flow with less vessel resistance. In addition, many veins of the body, particularly those of the limbs, contain valves that assist the unidirectional flow of blood toward the heart. This is critical because blood flow becomes sluggish in the extremities, as a result of the lower pressure and the effects of gravity.
Both arteries and veins have the same three distinct tissue layers, called tunics (from the Latin term tunica), for the garments first worn by ancient Romans; the term tunic is also used for some modern garments. From the most interior layer to the outer, these tunics are the tunica intima, the tunica media, and the tunica externa (Figure 2 and Table 1).
Tunica Intima: The tunica intima (also called the tunica interna) is composed of epithelial and connective tissue layers. Lining the tunica intima is the specialized simple squamous epithelium called the endothelium, which is continuous throughout the entire vascular system, including the lining of the chambers of the heart. Damage to this endothelial lining and exposure of blood to the collagenous fibres beneath is one of the primary causes of clot formation. Until recently, the endothelium was viewed simply as the boundary between the blood in the lumen and the walls of the vessels. Recent studies, however, have shown that it is physiologically critical to such activities as helping to regulate capillary exchange and altering blood flow. The endothelium releases local chemicals called endothelins that can constrict the smooth muscle within the walls of the vessel to increase blood pressure. Uncompensated overproduction of endothelins may contribute to hypertension (high blood pressure) and cardiovascular disease.
Next to the endothelium is the basement membrane, or basal lamina, that effectively binds the endothelium to the connective tissue. The basement membrane provides strength while maintaining flexibility, and it is permeable, allowing materials to pass through it. The thin outer layer of the tunica intima contains a small amount of areolar connective tissue that consists primarily of elastic fibres to provide the vessel with additional flexibility; it also contains some collagenous fibres to provide additional strength.
In larger arteries, there is also a thick, distinct layer of elastic fibers known as the internal elastic membrane (also called the internal elastic lamina) at the boundary with the tunica media. Like the other components of the tunica intima, the internal elastic membrane provides structure while allowing the vessel to stretch. It is permeated with small openings that allow exchange of materials between the tunics. The internal elastic membrane is not apparent in veins. In addition, many veins, particularly in the lower limbs, contain valves formed by sections of thickened endothelium that are reinforced with connective tissue, extending into the lumen.
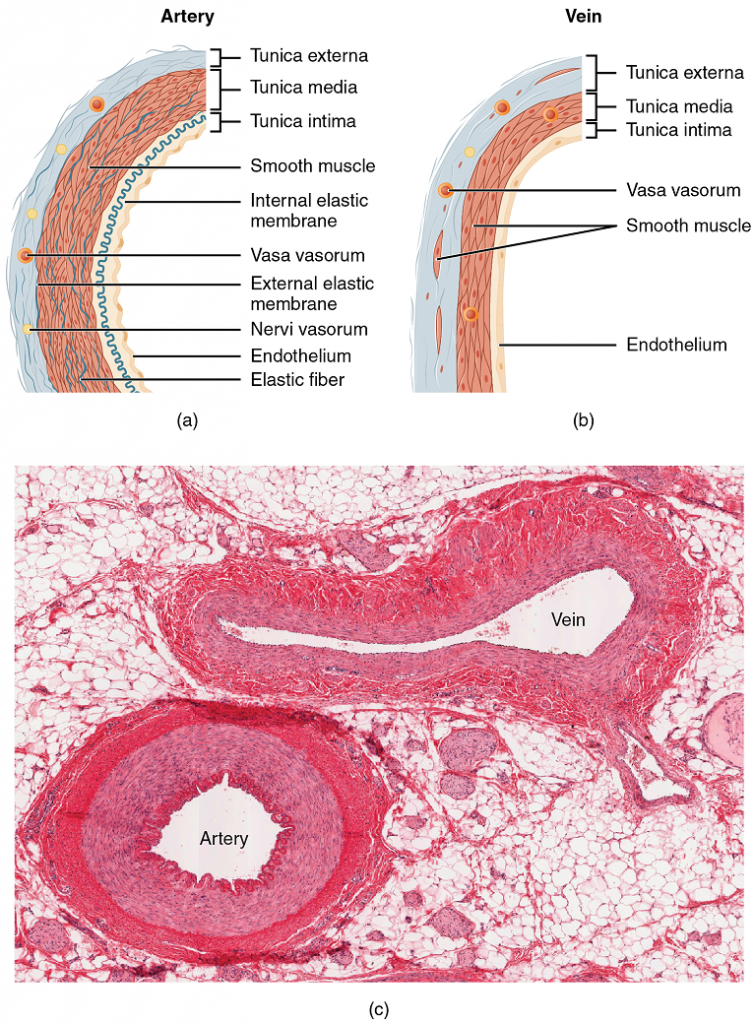
Tunica Media: The tunica media is the substantial middle layer of the vessel wall (Figure 2). It is generally the thickest layer in arteries, and it is much thicker in arteries than it is in veins. The tunica media consists of layers of smooth muscle supported by connective tissue that is primarily made up of elastic fibers, most of which are arranged in circular sheets. Toward the outer portion of the tunic, there are also layers of longitudinal muscle. Contraction and relaxation of the circular muscles decrease and increase the diameter of the vessel lumen, respectively. Specifically, in arteries, vasoconstriction decreases blood flow as the smooth muscle in the walls of the tunica media contracts, making the lumen narrower and increasing blood pressure. Similarly, vasodilation increases blood flow as the smooth muscle relaxes, allowing the lumen to widen and blood pressure to drop.
The smooth muscle layers of the tunica media are supported by a framework of collagenous fibres that also binds the tunica media to the inner and outer tunics. Along with the collagenous fibers are large numbers of elastic fibres that appear as wavy lines in prepared slides.
Arteries | Veins | Capillaries | |
---|---|---|---|
General appearance | Thick walls with small lumens | Thin walls with large lumens | Very (microscopically) thin walls and very small lumens |
Generally appear rounded | Generally appear flattened | Generally round | |
Tunica intima | Endothelium usually appears wavy due to constriction of smooth muscle | Endothelium appears smooth | Endothelium appears smooth |
Internal elastic membrane present in larger vessels | Internal elastic membrane absent | Internal elastic membrane absent | |
Tunica media | Normally the thickest layer in arteries | Normally thinner than the tunica externa | Tunica media absent |
Smooth muscle cells and elastic fibers predominate (exact proportions vary with distance from the heart) | Smooth muscle cells and collagenous fibres predominate | ||
External elastic membrane present in larger vessels | External elastic membrane absent | ||
Nervi vasorum and vasa vasorum present | |||
Tunica externa | Normally thinner than tunica media in all but the largest arteries | Normally the thickest layer in veins | Tunica externa absent |
Collagenous and elastic fibres | Collagenous and smooth fibres predominate | ||
Nervi vasorum and vasa vasorum present | Nervi vasorum and vasa vasorum present |
Tunica Externa: The outer tunic, the tunica externa (also called the tunica adventitia), is a substantial sheath of connective tissue composed primarily of collagenous fibres. Some bands of elastic fibres are found here as well. The tunica externa in veins also contains groups of smooth muscle fibres. This is normally the thickest tunic in veins and may be thicker than the tunica media in some larger arteries.
Arteries
An artery is a blood vessel that conducts blood away from the heart. All arteries have relatively thick walls that can withstand the high pressure of blood ejected from the heart.
Arterioles
An arteriole is a very small artery that leads to a capillary. Arterioles have the same three tunics as the larger vessels, but the thickness of each is greatly diminished. The critical endothelial lining of the tunica intima is intact. The tunica media is restricted to one or two smooth muscle cell layers in thickness. The tunica externa remains but is very thin (Figure 39).
The importance of the arterioles is that they will be the primary site of both resistance and regulation of blood pressure. The precise diameter of the lumen of an arteriole at any given moment is determined by neural and chemical controls, and vasoconstriction and vasodilation in the arterioles are the primary mechanisms for distribution of blood flow.
Capillaries
A capillary is a microscopic channel that supplies blood to the tissues themselves, a process called perfusion. Exchange of gases and other substances occurs in the capillaries between the blood and the surrounding cells and their tissue fluid (interstitial fluid). The diameter of a capillary lumen is from 5-10 μm; the smallest are just barely wide enough for an erythrocyte to squeeze through. Flow through capillaries is often described as microcirculation.
Unlike the walls of veins and arteries, the wall of a capillary consists of an endothelial layer surrounded by a basement membrane with occasional smooth muscle fibres. There is some variation in wall structure: in a large capillary, several endothelial cells bordering each other may line the lumen; in a small capillary, there may be only a single cell layer that wraps around to contact itself.
Venules
A venule is an extremely small vein, generally 8–100 μm in diameter. Postcapillary venules join multiple capillaries exiting from a capillary bed. Multiple venules join to form veins. The walls of venules consist of endothelium, a thin middle layer with a few muscle cells and elastic fibers, plus an outer layer of connective tissue fibers that constitute a very thin tunica externa. Venules as well as capillaries are the primary sites of emigration or diapedesis, in which the leukocytes adhere to the endothelial lining of the vessels and then squeeze through adjacent cells to enter the tissue fluid.
Veins
A vein is a blood vessel that conducts blood toward the heart. Compared to arteries, veins are thin-walled vessels with large and irregular lumens (Figure 42). Because they are low-pressure vessels, larger veins are commonly equipped with valves that promote the unidirectional flow of blood toward the heart and prevent backflow toward the capillaries caused by the inherent low blood pressure in veins as well as the pull of gravity. Table 2 compares the features of arteries and veins.
Arteries | Veins | |
---|---|---|
Direction of blood flow | Conducts blood away from the heart | Conducts blood toward the heart |
General appearance | Rounded | Irregular, often collapsed |
Pressure | High | Low |
Wall thickness | Thick | Thin |
Relative oxygen concentration | Higher in systemic arteries; lower in pulmonary arteries | Lower in systemic veins; higher in pulmonary venis |
Valves | Not present | Present most commonly in limbs and in veins inferior to the heart |
Part 2: Capillary Exchange
The primary purpose of the cardiovascular system is to circulate gases, nutrients, wastes, and other substances to and from the cells of the body. Small molecules, such as gases, lipids, and lipid-soluble molecules, can diffuse directly through the membranes of the endothelial cells of the capillary wall. Glucose, amino acids, and ions—including sodium, potassium, calcium, and chloride—use transporters to move through specific channels in the membrane by facilitated diffusion. Glucose, ions, and larger molecules may also leave the blood through intercellular clefts. Larger molecules can pass through the pores of fenestrated capillaries, and even large plasma proteins can pass through the great gaps in the sinusoid capillaries. Some large proteins in blood plasma can move into and out of the endothelial cells packaged within vesicles by endocytosis and exocytosis. Water moves by osmosis.
Part 3: Blood Flow, Blood Pressure, and Resistance
Blood flow refers to the movement of blood through a vessel, tissue, or organ, and is usually expressed in terms of volume of blood per unit of time. It is initiated by the contraction of the ventricles of the heart. Ventricular contraction ejects blood into the major arteries, resulting in flow from regions of higher pressure to regions of lower pressure, as blood encounters smaller arteries and arterioles, then capillaries, then the venules and veins of the venous system. This section discusses a number of critical variables that contribute to blood flow throughout the body. It also discusses the factors that impede or slow blood flow, a phenomenon known as resistance.
As noted earlier, hydrostatic pressure is the force exerted by a fluid due to gravitational pull, usually against the wall of the container in which it is located. One form of hydrostatic pressure is blood pressure, the force exerted by blood upon the walls of the blood vessels or the chambers of the heart. Blood pressure may be measured in capillaries and veins, as well as the vessels of the pulmonary circulation; however, the term blood pressure without any specific descriptors typically refers to systemic arterial blood pressure—that is, the pressure of blood flowing in the arteries of the systemic circulation. In clinical practice, this pressure is measured in mm Hg and is usually obtained using the brachial artery of the arm.
Components of Arterial Blood Pressure
Arterial blood pressure in the larger vessels consists of several distinct components (Figure 3): systolic and diastolic pressures, pulse pressure, and mean arterial pressure.
Systolic and Diastolic Pressures: When systemic arterial blood pressure is measured, it is recorded as a ratio of two numbers (e.g., 120/80 is a normal adult blood pressure), expressed as systolic pressure over diastolic pressure. The systolic pressure is the higher value (typically around 120 mm Hg) and reflects the arterial pressure resulting from the ejection of blood during ventricular contraction, or systole. The diastolic pressure is the lower value (usually about 80 mm Hg) and represents the arterial pressure of blood during ventricular relaxation, or diastole.
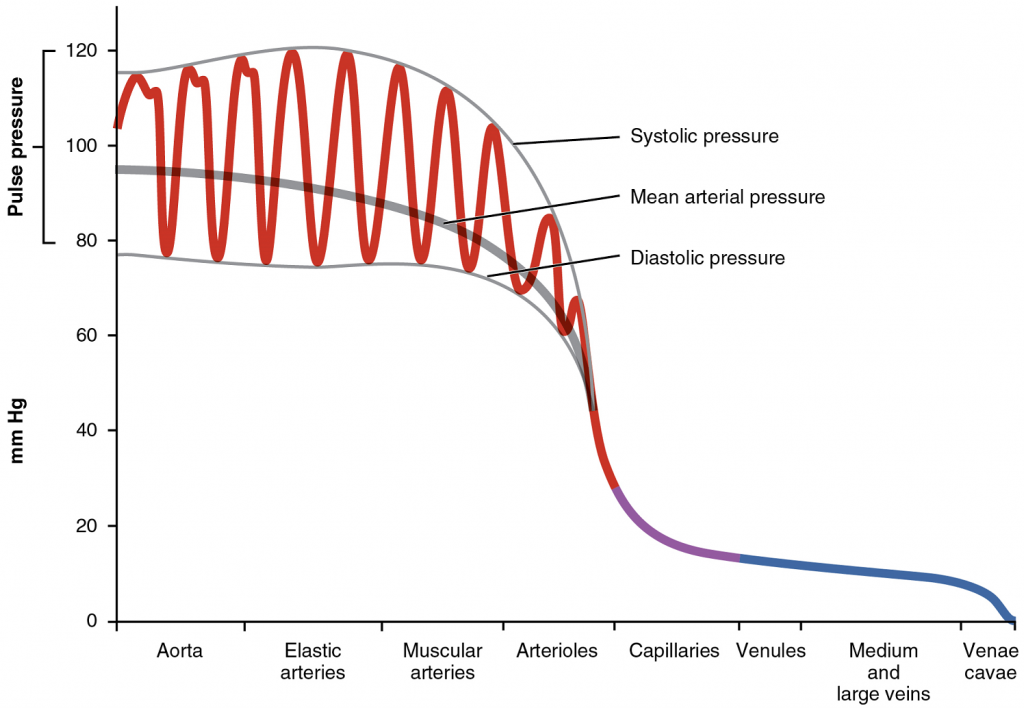
Mean Arterial Pressure: Mean arterial pressure (MAP) represents the “average” pressure of blood in the arteries, that is, the average force driving blood into vessels that serve the tissues. Mean is a statistical concept and is calculated by taking the sum of the values divided by the number of values. Although complicated to measure directly and complicated to calculate, MAP can be approximated by adding the diastolic pressure to one-third of the pulse pressure or systolic pressure minus the diastolic pressure:
MAP = diastolic BP + ((systolic-diastolic BP) / 3)
Normally, the MAP falls within the range of 70–110 mm Hg. If the value falls below 60 mm Hg for an extended time, blood pressure will not be high enough to ensure circulation to and through the tissues, which results in ischemia, or insufficient blood flow. A condition called hypoxia, inadequate oxygenation of tissues, commonly accompanies ischemia. The term hypoxemia refers to low levels of oxygen in systemic arterial blood.
Measurement of Blood Pressure: Blood pressure is one of the critical parameters measured on virtually every patient in every healthcare setting. The technique used today was developed more than 100 years ago by a pioneering Russian physician, Dr. Nikolai Korotkoff. Turbulent blood flow through the vessels can be heard as a soft ticking while measuring blood pressure; these sounds are known as Korotkoff sounds. The technique of measuring blood pressure requires the use of a sphygmomanometer (a blood pressure cuff attached to a measuring device) and a stethoscope. The technique is as follows:
- The clinician wraps an inflatable cuff tightly around the patient’s arm at about the level of the heart.
- The clinician squeezes a rubber pump to inject air into the cuff, raising pressure around the artery and temporarily cutting off blood flow into the patient’s arm.
- The clinician places the stethoscope on the patient’s antecubital region and, while gradually allowing air within the cuff to escape, listens for the Korotkoff sounds.
The first sound heard through the stethoscope—the first Korotkoff sound—indicates systolic pressure. As more air is released from the cuff, blood is able to flow freely through the brachial artery and all sounds disappear. The point at which the last sound is heard is recorded as the patient’s diastolic pressure.
Pulse
After blood is ejected from the heart, elastic fibres in the arteries help maintain a high-pressure gradient as they expand to accommodate the blood, then recoil. This expansion and recoiling effect, known as the pulse, can be palpated manually or measured electronically. Although the effect diminishes over distance from the heart, elements of the systolic and diastolic components of the pulse are still evident down to the level of the arterioles.
Because pulse indicates heart rate, it is measured clinically to provide clues to a patient’s state of health. It is recorded as beats per minute. Both the rate and the strength of the pulse are important clinically. A high or irregular pulse rate can be caused by physical activity or other temporary factors, but it may also indicate a heart condition. The pulse strength indicates the strength of ventricular contraction and cardiac output. If the pulse is strong, then systolic pressure is high. If it is weak, systolic pressure has fallen, and medical intervention may be warranted.
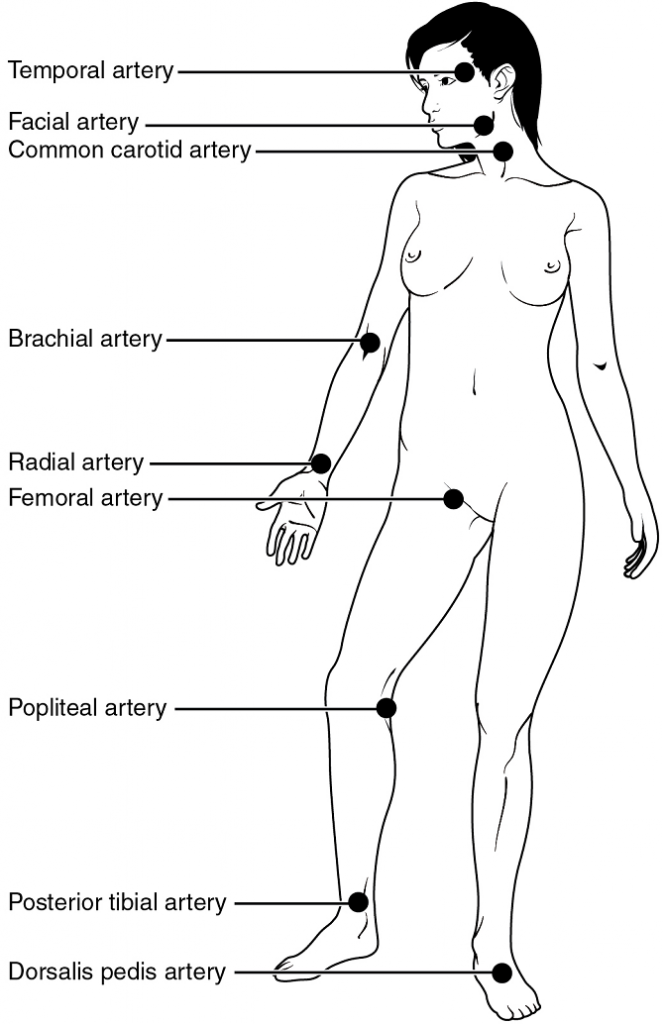
Pulse can be palpated manually by placing the tips of the fingers across an artery that runs close to the body surface and pressing lightly. While this procedure is normally performed using the radial artery in the wrist or the common carotid artery in the neck, any superficial artery that can be palpated may be used (Figure 4). Common sites to find a pulse include temporal and facial arteries in the head, brachial arteries in the upper arm, femoral arteries in the thigh, popliteal arteries behind the knees, posterior tibial arteries near the medial tarsal regions, and dorsalis pedis arteries in the feet. A variety of commercial electronic devices are also available to measure pulse.
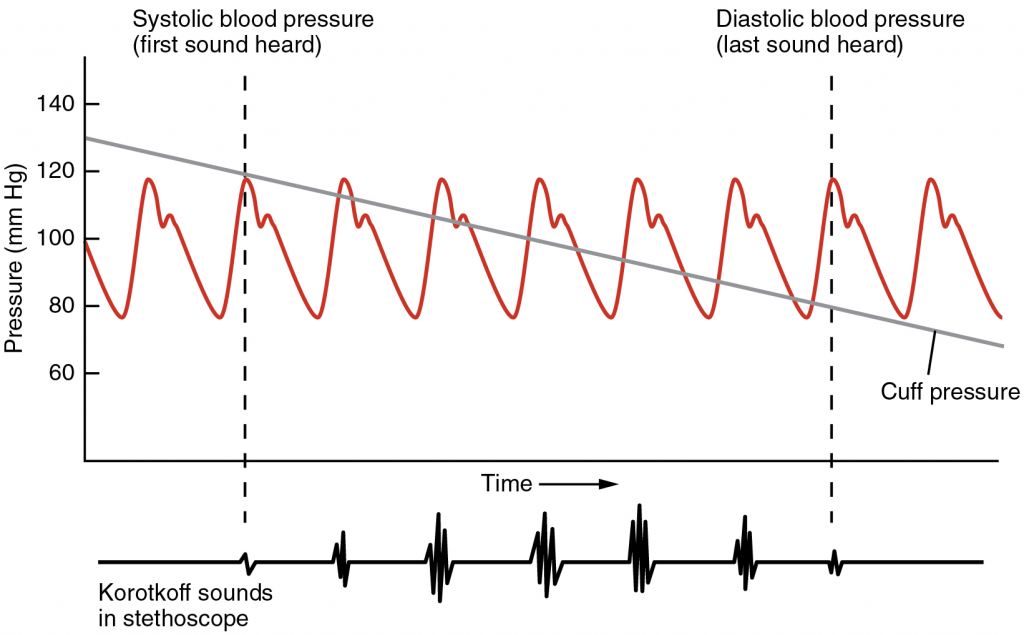
Variables Affecting Blood Flow and Blood Pressure
Five variables influence blood flow and blood pressure:
- Cardiac output
- Compliance
- Volume of the blood
- Viscosity of the blood
- Blood vessel length and diameter
Recall that blood moves from higher pressure to lower pressure. It is pumped from the heart into the arteries at high pressure. If you increase pressure in the arteries (afterload), and cardiac function does not compensate, blood flow will actually decrease. In the venous system, the opposite relationship is true. Increased pressure in the veins does not decrease flow as it does in arteries, but actually increases flow. Since pressure in the veins is normally relatively low, for blood to flow back into the heart, the pressure in the atria during atrial diastole must be even lower. It normally approaches zero, except when the atria contract (Figure 5).
Cardiac Output: Cardiac output is the measurement of blood flow from the heart through the ventricles, and is usually measured in liters per minute. Any factor that causes cardiac output to increase, by elevating heart rate or stroke volume or both, will elevate blood pressure and promote blood flow. These factors include sympathetic stimulation, the catecholamines epinephrine and norepinephrine, thyroid hormones, and increased calcium ion levels. Conversely, any factor that decreases cardiac output, by decreasing heart rate or stroke volume or both, will decrease arterial pressure and blood flow. These factors include parasympathetic stimulation, elevated or decreased potassium ion levels, decreased calcium levels, anoxia, and acidosis.
Compliance: Compliance is the ability of any compartment to expand to accommodate increased content. A metal pipe, for example, is not compliant, whereas a balloon is. The greater the compliance of an artery, the more effectively it is able to expand to accommodate surges in blood flow without increased resistance or blood pressure. Veins are more compliant than arteries and can expand to hold more blood. When vascular disease causes stiffening of arteries, compliance is reduced and resistance to blood flow is increased. The result is more turbulence, higher pressure within the vessel, and reduced blood flow. This increases the work of the heart.
Blood Volume: The relationship between blood volume, blood pressure, and blood flow is intuitively obvious. Water may merely trickle along a creek bed in a dry season, but rush quickly and under great pressure after a heavy rain. Similarly, as blood volume decreases, pressure and flow decrease. As blood volume increases, pressure and flow increase.
Blood Viscosity: Viscosity is the thickness of fluids that affects their ability to flow. Clean water, for example, is less viscous than mud. The viscosity of blood is directly proportional to resistance and inversely proportional to flow; therefore, any condition that causes viscosity to increase will also increase resistance (and therefore blood pressure) and decrease flow. For example, imagine sipping milk, then a milkshake, through the same size straw. You experience more resistance and therefore less flow from the milkshake. Conversely, any condition that causes viscosity to decrease (such as when the milkshake melts) will decrease resistance and increase flow.
Normally the viscosity of blood does not change over short periods of time. The two primary determinants of blood viscosity are the formed elements and plasma proteins. Since the vast majority of formed elements are erythrocytes, any condition affecting erythropoiesis, such as polycythemia or anemia, can alter viscosity. Viscosity generally increases with increasing numbers of formed elements relative to the amount of plasma. If the concentration of proteins in the plasma is increased, this would also increase viscosity. Since most plasma proteins are produced by the liver, any condition affecting liver function can also change the viscosity and therefore affect blood flow. Liver abnormalities include hepatitis, cirrhosis, alcohol damage, and drug toxicities. While leukocytes and platelets are normally a small component of the formed elements, there are some rare conditions in which there is such a great overproduction of these that viscosity increases.
Vessel Length and Diameter: The length of a vessel is directly proportional to its resistance: the longer the vessel, the greater the resistance and the lower the flow. As with blood volume, this makes intuitive sense, since the increased surface area of the vessel will impede the flow of blood. Likewise, if the vessel is shortened, the resistance will decrease and flow will increase.
In contrast to length, the diameter of blood vessels changes throughout the body, according to the type of vessel, as we discussed earlier. The diameter of any given vessel may also change frequently throughout the day in response to neural and chemical signals that trigger vasodilation and vasoconstriction. The vascular tone of the vessel is the contractile state of the smooth muscle and the primary determinant of diameter, and thus of resistance and flow. The effect of vessel diameter on resistance is inverse: Given the same volume of blood, an increased diameter means there is less blood contacting the vessel wall, thus lower friction and lower resistance, subsequently increasing flow. A decreased diameter means more of the blood contacts the vessel wall, and resistance increases, subsequently decreasing flow.
Vasodilation and vasoconstriction of arterioles play more significant roles in regulating blood pressure than do the vasodilation and vasoconstriction of other vessels.
Venous System
The pumping action of the heart propels the blood into the arteries, from an area of higher pressure toward an area of lower pressure. If blood is to flow from the veins back into the heart, the pressure in the veins must be greater than the pressure in the atria of the heart. Two factors help maintain this pressure gradient between the veins and the heart. First, the pressure in the atria during diastole is very low, often approaching zero when the atria are relaxed (atrial diastole). Second, two physiologic “pumps” increase pressure in the venous system. The use of the term “pump” implies a physical device that speeds flow. These physiological pumps are less obvious.
Skeletal Muscle Pump: In many body regions, the pressure within the veins can be increased by the contraction of the surrounding skeletal muscle. This mechanism, known as the skeletal muscle pump (Figure 6), helps the lower-pressure veins counteract the force of gravity, increasing pressure to move blood back to the heart. As leg muscles contract, for example during walking or running, they exert pressure on nearby veins with their numerous one-way valves. This increased pressure causes blood to flow upward, opening valves superior to the contracting muscles so blood flows through. Simultaneously, valves inferior to the contracting muscles close; thus, blood should not seep back downward toward the feet. Military recruits are trained to flex their legs slightly while standing at attention for prolonged periods. Failure to do so may allow blood to pool in the lower limbs rather than returning to the heart. Consequently, the brain will not receive enough oxygenated blood, and the individual may lose consciousness.
Respiratory Pump: The respiratory pump aids blood flow through the veins of the thorax and abdomen. During inhalation, the volume of the thorax increases, largely through the contraction of the diaphragm, which moves downward and compresses the abdominal cavity. The elevation of the chest caused by the contraction of the external intercostal muscles also contributes to the increased volume of the thorax. The volume increase causes air pressure within the thorax to decrease, allowing us to inhale. Additionally, as air pressure within the thorax drops, blood pressure in the thoracic veins also decreases, falling below the pressure in the abdominal veins. This causes blood to flow along its pressure gradient from veins outside the thorax, where pressure is higher, into the thoracic region, where pressure is now lower. This in turn promotes the return of blood from the thoracic veins to the atria. During exhalation, when air pressure increases within the thoracic cavity, pressure in the thoracic veins increases, speeding blood flow into the heart while valves in the veins prevent blood from flowing backward from the thoracic and abdominal veins. Also notice that, as blood moves from venules to veins, the average blood pressure drops.
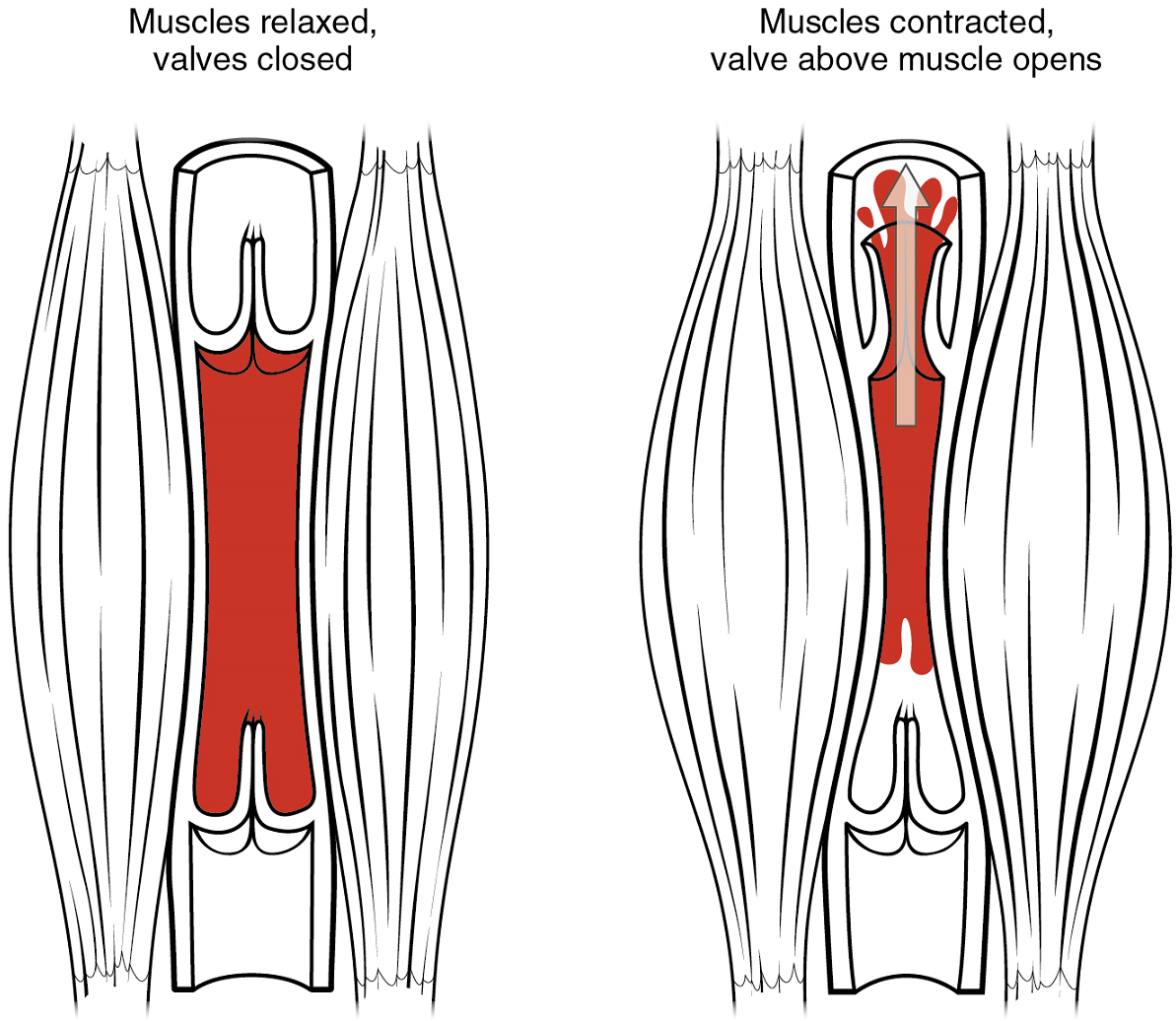
Part 4: Homeostatic Regulation of the Vascular System
To maintain homeostasis in the cardiovascular system and provide adequate blood to the tissues, blood flow must be redirected continually to the tissues as they become more active. In a very real sense, the cardiovascular system engages in resource allocation, because there is not enough blood flow to distribute blood equally to all tissues simultaneously. For example, when an individual is exercising, more blood will be directed to skeletal muscles, the heart, and the lungs. Following a meal, more blood is directed to the digestive system. Only the brain receives a more or less constant supply of blood whether you are active, resting, thinking, or engaged in any other activity.
Table 3 provides the distribution of systemic blood at rest and during exercise. Although most of the data appears logical, the values for the distribution of blood to the integument may seem surprising. During exercise, the body distributes more blood to the body surface where it can dissipate the excess heat generated by increased activity into the environment. Three homeostatic mechanisms ensure adequate blood flow, blood pressure, distribution, and ultimately perfusion: neural, endocrine, and autoregulatory mechanisms (Figure 7).
Organ | Resting (mL/min) | Mild exercise (mL/min) | Maximal exercise (mL/min) |
---|---|---|---|
Skeletal muscle | 1200 | 4500 | 12,500 |
Heart | 250 | 350 | 750 |
Brain | 750 | 750 | 750 |
Integument | 500 | 1500 | 1900 |
Kidney | 1100 | 900 | 600 |
Gastrointestinal | 1400 | 1100 | 600 |
Others (e.g., liver, spleen) | 600 | 400 | 400 |
Total | 5800 | 9500 | 17,500 |
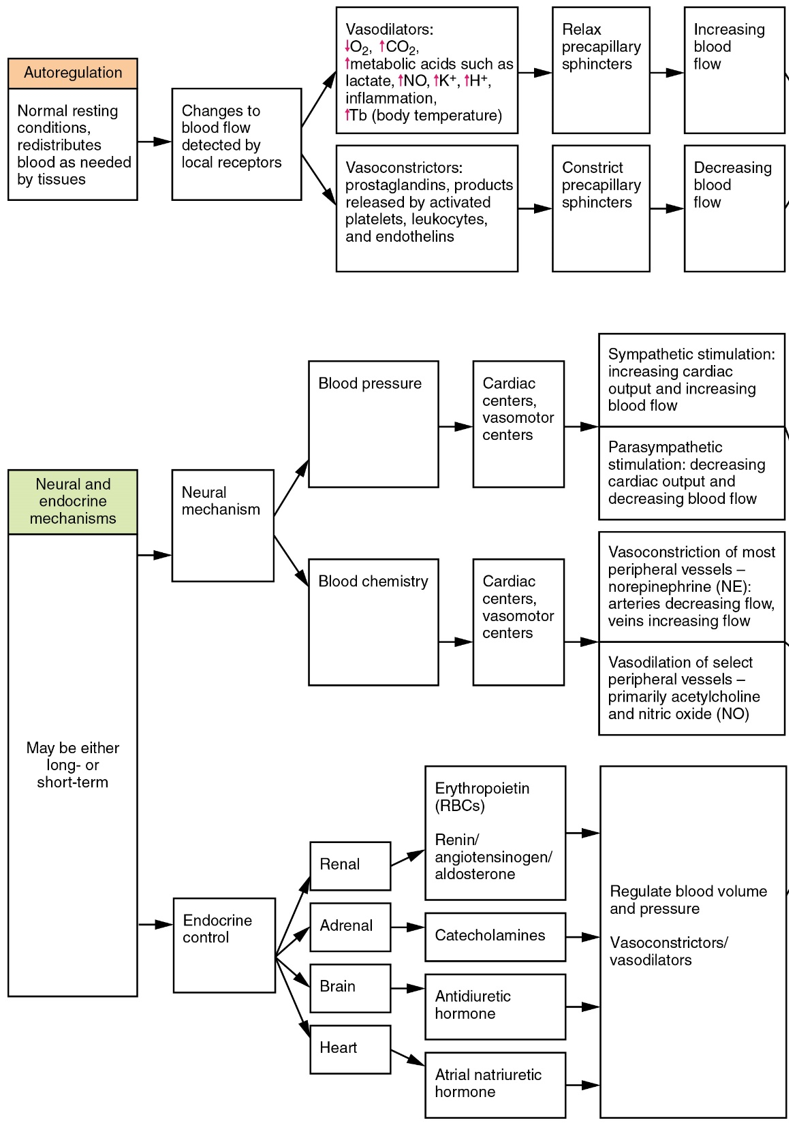
Neural Regulation
The nervous system plays a critical role in the regulation of vascular homeostasis. The primary regulatory sites include the cardiovascular centres in the brain that control both cardiac and vascular functions. In addition, more generalized neural responses from the limbic system and the autonomic nervous system are factors.
The Cardiovascular Centres in the Brain
Neurological regulation of blood pressure and flow depends on the cardiovascular centres located in the medulla oblongata. This cluster of neurons responds to changes in blood pressure as well as blood concentrations of oxygen, carbon dioxide, and hydrogen ions. The cardiovascular centre contains three distinct components:
- The cardioacceleratory centre stimulates cardiac function by regulating heart rate and stroke volume via sympathetic stimulation from the cardiac accelerator nerve.
- The cardioinhibitory centre slows cardiac function by decreasing heart rate via parasympathetic stimulation from the vagus nerve.
- The vasomotor centre controls vessel tone or contraction of the smooth muscle in the tunica media. Changes in diameter affect peripheral resistance, pressure, and flow, which affect cardiac output. The majority of these neurons act via the release of the neurotransmitter norepinephrine from sympathetic neurons.
Although each centre functions independently, they are not anatomically distinct.
There is also a small population of neurons that control vasodilation in the vessels of the brain and skeletal muscles by relaxing the smooth muscle fibres in the vessel tunics. Many of these are cholinergic neurons, that is, they release acetylcholine, which in turn stimulates the vessels’ endothelial cells to release nitric oxide (NO), which causes vasodilation. Others release norepinephrine that binds to β2 receptors. A few neurons release NO directly as a neurotransmitter.
Baroreceptor Reflexes
Baroreceptors are specialized stretch receptors located within thin areas of blood vessels and heart chambers that respond to the degree of stretch caused by the presence of blood. They send impulses to the cardiovascular centres to regulate blood pressure. Vascular baroreceptors are found primarily in sinuses (small cavities) within the aorta and carotid arteries: The aortic sinuses are found in the walls of the ascending aorta just superior to the aortic valve, whereas the carotid sinuses are in the base of the internal carotid arteries. There are also low-pressure baroreceptors located in the walls of the venae cavae and right atrium.
When blood pressure increases, the baroreceptors are stretched more tightly and initiate action potentials at a higher rate. At lower blood pressures, the degree of stretch is lower and the rate of firing is slower. When the cardiovascular centres in the medulla oblongata receives this input, they triggers a reflex that maintains homeostasis (Figure 8):
- When blood pressure rises too high, the baroreceptors fire at a higher rate and trigger parasympathetic stimulation of the heart. As a result, cardiac output falls. Sympathetic stimulation of the peripheral arterioles will also decrease, resulting in vasodilation. Combined, these activities cause blood pressure to fall.
- When blood pressure drops too low, the rate of baroreceptor firing decreases. This will trigger an increase in sympathetic stimulation of the heart, causing cardiac output to increase. It will also trigger sympathetic stimulation of the peripheral vessels, resulting in vasoconstriction. Combined, these activities cause blood pressure to rise.
The baroreceptors in the venae cavae and right atrium monitor blood pressure as the blood returns to the heart from the systemic circulation. Normally, blood flow into the aorta is the same as blood flow back into the right atrium. If blood is returning to the right atrium more rapidly than it is being ejected from the left ventricle, the atrial receptors will stimulate the cardiovascular centres to increase sympathetic firing and increase cardiac output until homeostasis is achieved. The opposite is also true. This mechanism is referred to as the atrial reflex.
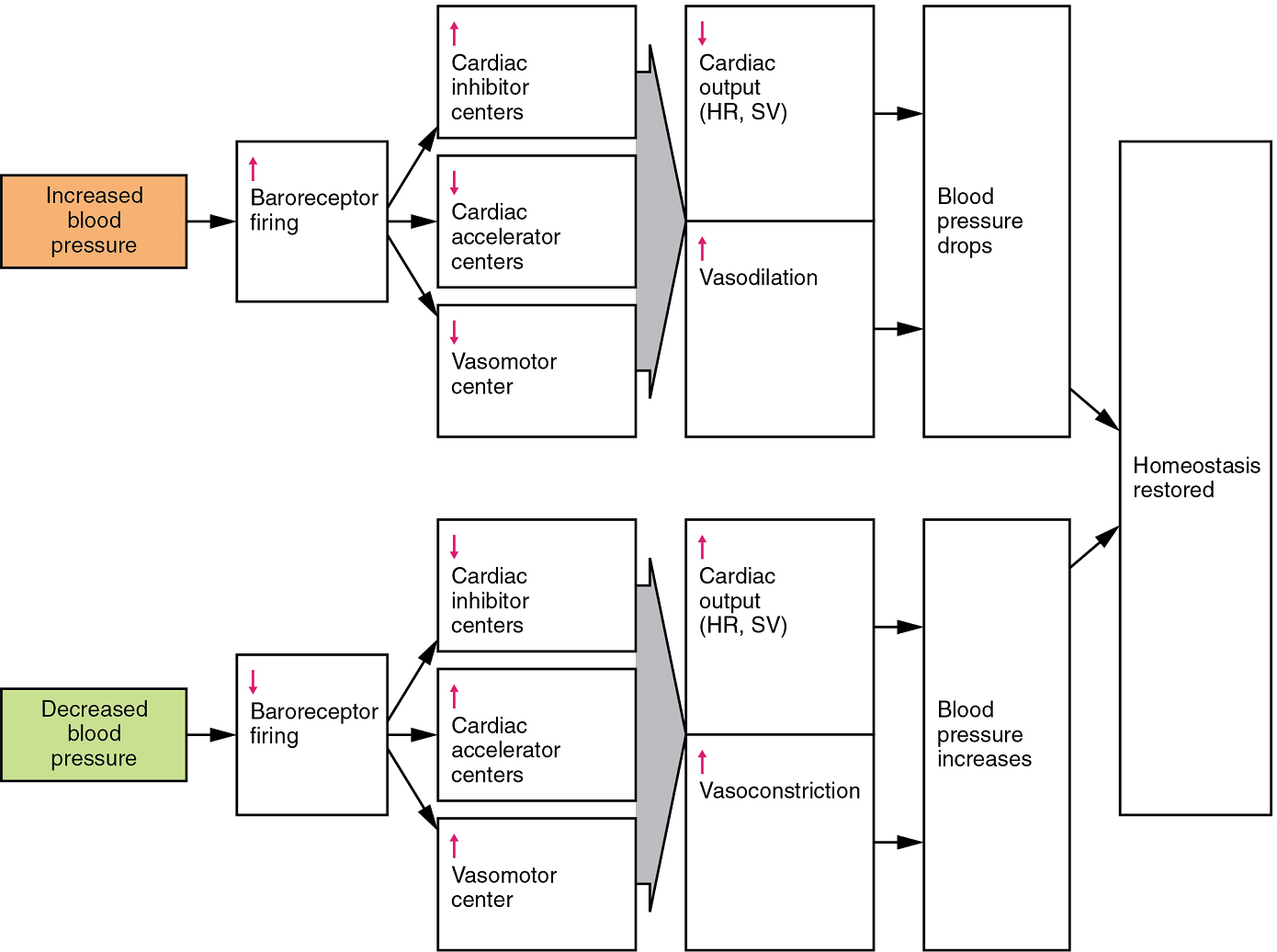
Chemoreceptor Reflexes: In addition to the baroreceptors are chemoreceptors that monitor levels of oxygen, carbon dioxide, and hydrogen ions (pH), and thereby contribute to vascular homeostasis. Chemoreceptors monitoring the blood are located in close proximity to the baroreceptors in the aortic and carotid sinuses. They signal the cardiovascular centres as well as the respiratory centres in the medulla oblongata.
Since tissues consume oxygen and produce carbon dioxide and acids as waste products, when the body is more active, oxygen levels fall and carbon dioxide levels rise as cells undergo cellular respiration to meet the energy needs of activities. This causes more hydrogen ions to be produced, causing the blood pH to drop. When the body is resting, oxygen levels are higher, carbon dioxide levels are lower, more hydrogen is bound, and pH rises.
The chemoreceptors respond to increasing carbon dioxide and hydrogen ion levels (falling pH) by stimulating the cardioacceleratory and vasomotor centres, increasing cardiac output and constricting peripheral vessels. The cardioinhibitory centre is suppressed. With falling carbon dioxide and hydrogen ion levels (increasing pH), the cardioinhibitory centre is stimulated, and the cardioacceleratory and vasomotor centres are suppressed, decreasing cardiac output and causing peripheral vasodilation. In order to maintain adequate supplies of oxygen to the cells and remove waste products such as carbon dioxide, it is essential that the respiratory system respond to changing metabolic demands. In turn, the cardiovascular system will transport these gases to the lungs for exchange, again in accordance with metabolic demands. This interrelationship of cardiovascular and respiratory control cannot be overemphasized.
Other neural mechanisms can also have affect cardiovascular function. These include the limbic system that links physiological responses to psychological stimuli, as well as generalized sympathetic and parasympathetic stimulation.
Endocrine Regulation
Endocrine control over the cardiovascular system involves the catecholamines, epinephrine and norepinephrine, as well as several hormones that interact with the kidneys in the regulation of blood volume.
Epinephrine and Norepinephrine: The catecholamines epinephrine and norepinephrine are released by the adrenal medulla, and enhance and extend the body’s sympathetic or “fight-or-flight” response (Figure 9). They increase heart rate and force of contraction, while temporarily constricting blood vessels to organs not essential for flight-or-fight responses and redirecting blood flow to the liver, muscles, and heart.
Antidiuretic Hormone: Antidiuretic hormone (ADH), also known as vasopressin, is secreted by the cells in the hypothalamus and transported via the hypothalamic-hypophyseal tracts to the posterior pituitary where it is stored until released upon nervous stimulation. The primary trigger prompting the hypothalamus to release antiduiretic hormone is increasing osmolarity of tissue fluid, usually in response to significant loss of blood volume (Figure 10). ADH signals its target cells in the kidneys to reabsorb more water, thus preventing the loss of additional fluid in the urine. This will increase overall fluid levels and help restore blood volume and pressure. In addition, antiduiretic hormone constricts peripheral vessels.
Renin-Angiotensin-Aldosterone Mechanism: The renin-angiotensin-aldosterone mechanism has a major effect upon the cardiovascular system (Figure 9). Renin is an enzyme, although because of its importance in the renin-angiotensin-aldosterone pathway, some sources identify it as a hormone. Specialized cells in the kidneys found in the juxtaglomerular apparatus respond to decreased blood flow by secreting renin into the blood. Renin converts the plasma protein angiotensinogen, which is produced by the liver, into its active form—angiotensin I. Angiotensin I circulates in the blood and is then converted into angiotensin II in the lungs. This reaction is catalyzed by the enzyme angiotensin-converting enzyme (ACE).
Angiotensin II is a powerful vasoconstrictor, greatly increasing blood pressure. It also stimulates the release of antiduiretic hormone and aldosterone, a hormone produced by the adrenal cortex. Aldosterone increases the reabsorption of sodium into the blood by the kidneys. Since water follows sodium, this increases the reabsorption of water. This in turn increases blood volume, raising blood pressure. Angiotensin II also stimulates the thirst centre in the hypothalamus, so an individual will likely consume more fluids, again increasing blood volume and pressure.
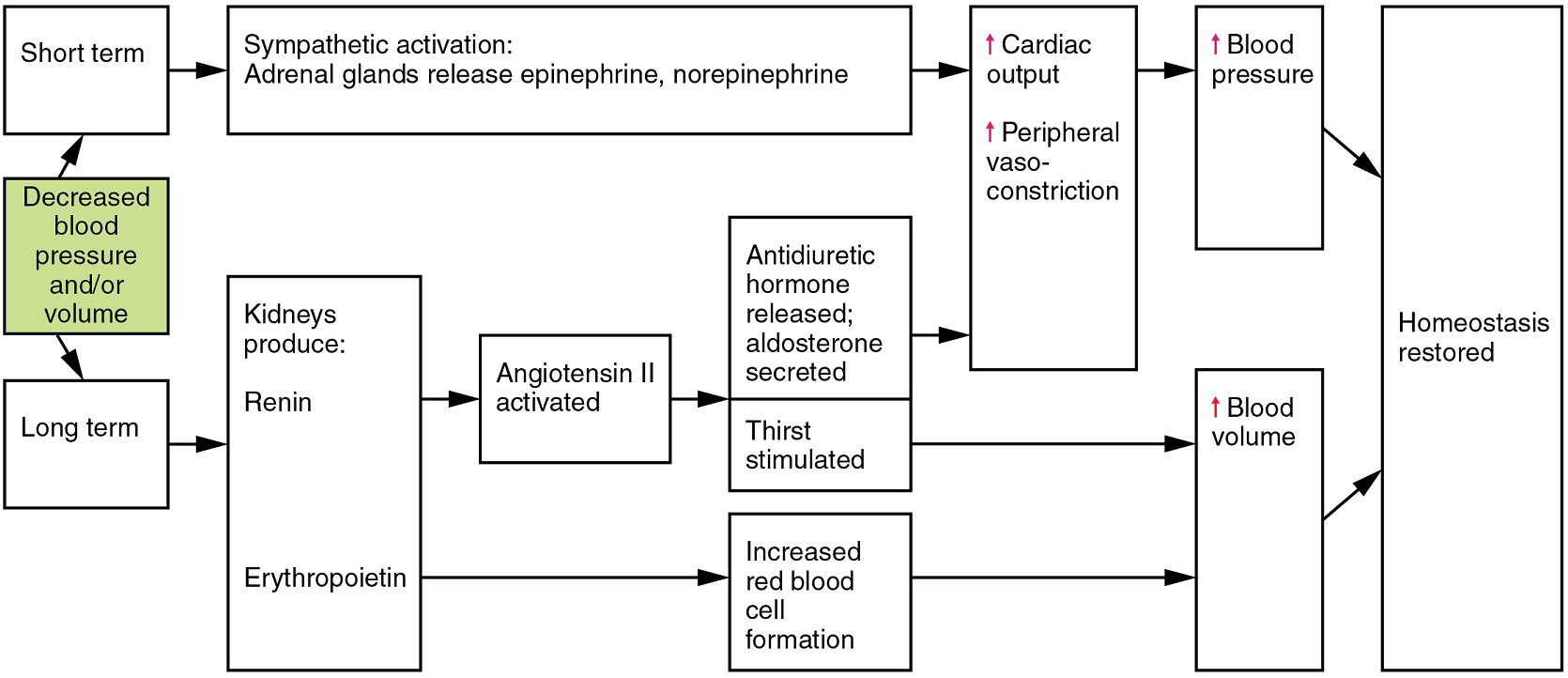
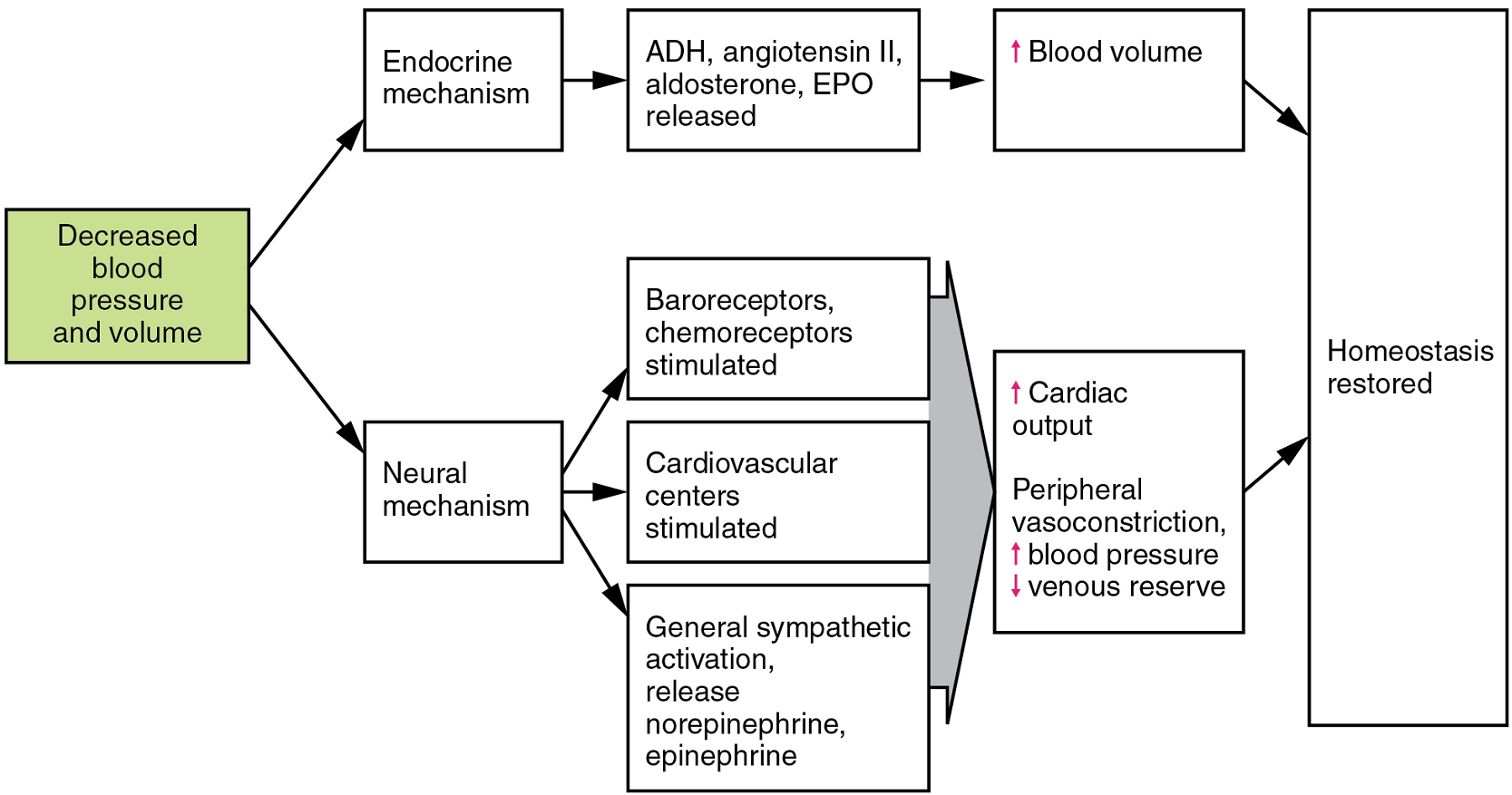
Erythropoietin: Erythropoietin (EPO) is released by the kidneys when blood flow and/or oxygen levels decrease. Erythropoietin stimulates the production of erythrocytes within the bone marrow. Erythrocytes are the major formed element of the blood and may contribute 40% or more to blood volume, a significant factor of viscosity, resistance, pressure, and flow. In addition, erythropoietin is a vasoconstrictor. Overproduction of erythropoietin or excessive intake of synthetic erythropoietin, often to enhance athletic performance, will increase viscosity, resistance, and pressure, and decrease flow in addition to its contribution as a vasoconstrictor.
Autoregulation of Perfusion
Autoregulation mechanisms require neither specialized nervous stimulation nor endocrine control. Rather, these are local, self-regulatory mechanisms that allow each region of tissue to adjust its blood flow, and thus its perfusion. These local mechanisms include chemical signals and myogenic controls.
Chemical Signals Involved in Autoregulation: Chemical signals work at the level of the precapillary sphincters to trigger either constriction or relaxation. Opening a precapillary sphincter allows blood to flow into that particular capillary, whereas constricting a precapillary sphincter temporarily shuts off blood flow to that region. The factors involved in regulating the precapillary sphincters include the following:
- Opening of the sphincter is triggered in response to decreased oxygen concentrations; increased carbon dioxide concentrations; increasing levels of lactic acid or other byproducts of cellular metabolism; increasing concentrations of potassium ions or hydrogen ions (falling pH); inflammatory chemicals such as histamines; and increased body temperature. These conditions in turn stimulate the release of NO, a powerful vasodilator, from endothelial cells.
- Contraction of the precapillary sphincter is triggered by the opposite levels of the regulators, which prompt the release of endothelins, powerful vasoconstricting peptides secreted by endothelial cells. Platelet secretions and certain prostaglandins may also trigger constriction.
Again, these factors alter tissue perfusion via their effects on the precapillary sphincter mechanism, which regulates blood flow to capillaries. Since the amount of blood is limited, not all capillaries can fill at once, so blood flow is allocated based upon the needs and metabolic state of the tissues as reflected in these parameters. Bear in mind, however, that dilation and constriction of the arterioles feeding the capillary beds is the primary control mechanism.
The Myogenic Response: The myogenic response is a reaction to the stretching of the smooth muscle in the walls of arterioles as changes in blood flow occur through the vessel. This may be viewed as a largely protective function against dramatic fluctuations in blood pressure and blood flow to maintain homeostasis. If perfusion of an organ is too low (ischemia), the tissue will experience low levels of oxygen (hypoxia). In contrast, excessive perfusion could damage the organ’s smaller and more fragile vessels. The myogenic response is a localized process that serves to stabilize blood flow in the capillary network that follows that arteriole. When blood flow is low, the vessel’s smooth muscle will be only minimally stretched. In response, it relaxes, allowing the vessel to dilate and thereby increase the movement of blood into the tissue. When blood flow is too high, the smooth muscle will contract in response to the increased stretch, prompting vasoconstriction that reduces blood flow.
Part 5: Circulatory Pathways
Virtually every cell, tissue, organ, and system in the body is impacted by the circulatory system. This includes the generalized and more specialized functions of transport of materials, capillary exchange, maintaining health by transporting leukocytes and various immunoglobulins (antibodies), hemostasis, regulation of body temperature, and helping to maintain acid-base balance. In addition to these shared functions, many systems enjoy a unique relationship with the circulatory system (Figure 11).
As you learn about the vessels of the systemic and pulmonary circuits, notice that many arteries and veins share the same names, parallel one another throughout the body, and are very similar on the right and left sides of the body. For example, you will find a pair of femoral arteries and a pair of femoral veins, with one vessel on each side of the body. In contrast, some vessels closer to the midline of the body, such as the aorta, are unique. Another phenomenon that can make the study of vessels challenging is that names of vessels can change with location. Like a street that changes name as it passes through an intersection, an artery or vein can change names as it passes an anatomical landmark. For example, the left subclavian artery becomes the axillary artery as it passes through the body wall and into the axillary region, and then becomes the brachial artery as it flows from the axillary region into the upper arm (or brachium).
Pulmonary Circulation
Recall that blood returning from the systemic circuit enters the right atrium (Figure 12) via the superior and inferior venae cavae and the coronary sinus, which drains the blood supply of the heart muscle. These vessels will be described more fully later in this section. This blood is relatively low in oxygen and relatively high in carbon dioxide, since much of the oxygen has been extracted for use by the tissues and the waste gas carbon dioxide was picked up to be transported to the lungs for elimination. From the right atrium, blood moves into the right ventricle, which pumps it to the lungs for gas exchange. This system of vessels is referred to as the pulmonary circuit.
The single vessel exiting the right ventricle is the pulmonary trunk. At the base of the pulmonary trunk is the pulmonary semilunar valve, which prevents backflow of blood into the right ventricle during ventricular diastole. As the pulmonary trunk reaches the superior surface of the heart, it curves posteriorly and rapidly bifurcates (divides) into two branches, a left and a right pulmonary artery. To prevent confusion between these vessels, it is important to refer to the vessel exiting the heart as the pulmonary trunk, rather than also calling it a pulmonary artery.
The pulmonary arteries in turn branch many times within the lung, forming a series of smaller arteries and arterioles that eventually lead to the pulmonary capillaries. The pulmonary capillaries surround lung structures known as alveoli that are the sites of oxygen and carbon dioxide exchange.
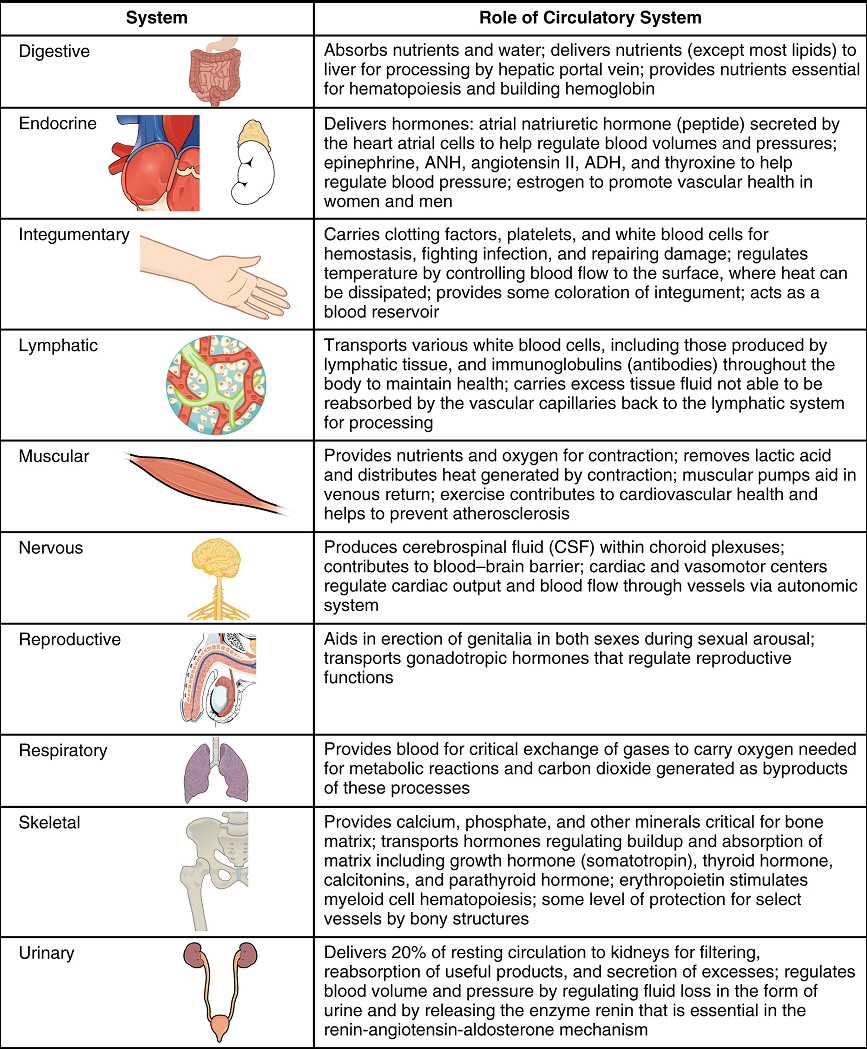
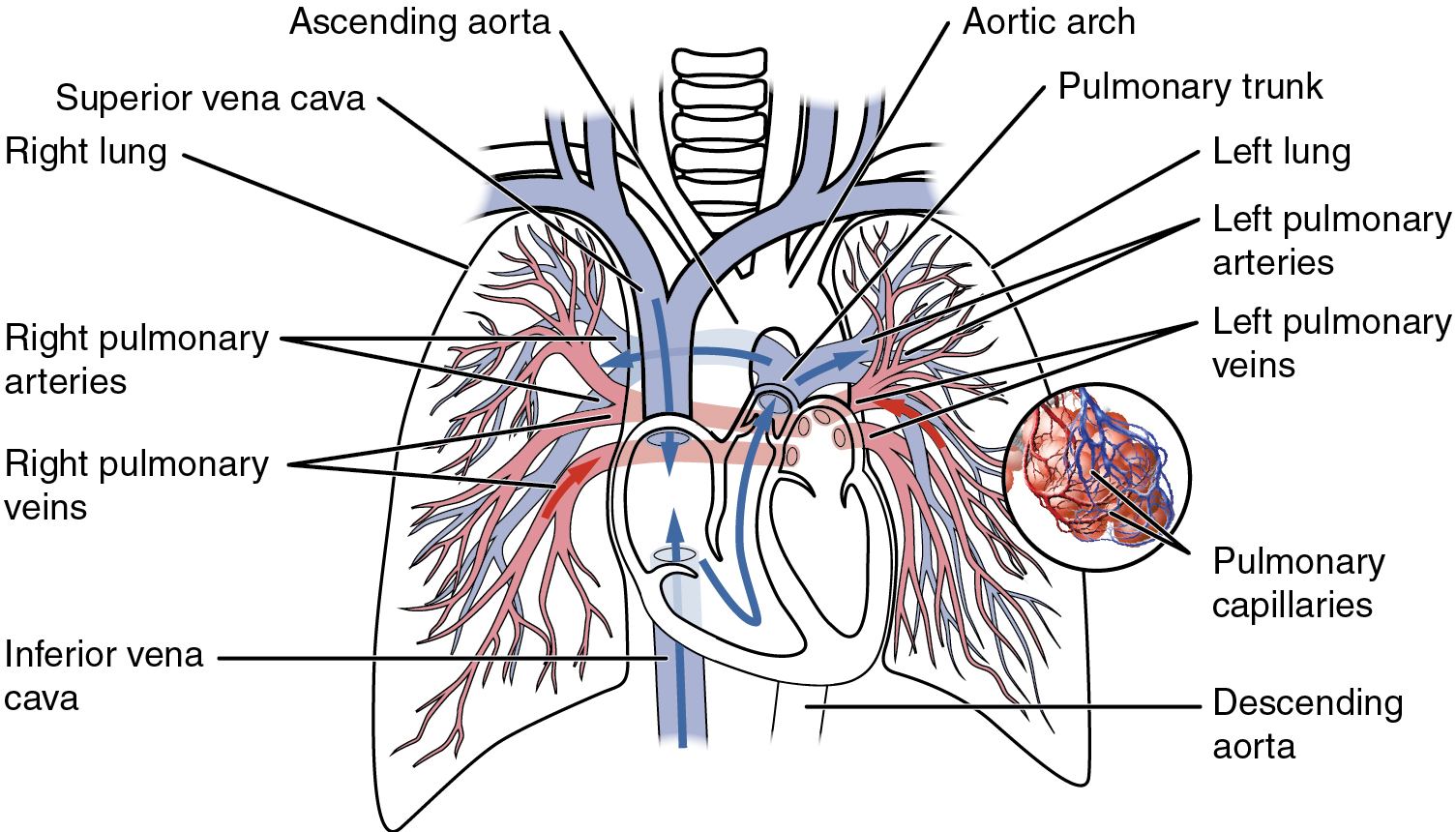
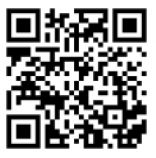
Once gas exchange is completed, oxygenated blood flows from the pulmonary capillaries into a series of pulmonary venules that eventually lead to a series of larger pulmonary veins. Four pulmonary veins, two on the left and two on the right, return blood to the left atrium. At this point, the pulmonary circuit is complete. Table 4 defines the major arteries and veins of the pulmonary circuit discussed in the text.
Overview of Systemic Arteries
Blood relatively high in oxygen concentration is returned from the pulmonary circuit to the left atrium via the four pulmonary veins. From the left atrium, blood moves into the left ventricle, which pumps blood into the aorta. The aorta and its branches—the systemic arteries—send blood to virtually every organ of the body (Figure 41).
Vessel | Description |
---|---|
Pulmonary trunk | Single large vessel exiting the right ventricle (divides to form the right and left pulmonary arteries) |
Pulmonary arteries (left pulmonary artery, right pulmonary artery) | Two vessels that form from the pulmonary trunk and lead to smaller arterioles and eventually to the pulmonary capillaries |
Pulmonary veins (left superior pulmonary vein, left inferior pulmonary vein, right superior pulmonary vein, right inferior pulmonary vein) | Two sets of paired vessels (one pair from each side) that are formed from venules, leading blood away from the pulmonary capillaries to flow into the left atrium |
The Aorta
The aorta is the largest artery in the body (Figure 13). It arises from the left ventricle and eventually descends to the abdominal region, where it bifurcates at the level of the fourth lumbar vertebra into the two common iliac arteries. The aorta consists of the ascending aorta, the aortic arch, and the descending aorta (Table 5) which passes through the diaphragm, a landmark that divides into the superior thoracic and inferior abdominal components. Arteries originating from the aorta ultimately distribute blood to virtually all tissues of the body. At the base of the aorta is the aortic semilunar valve that prevents backflow of blood into the left ventricle while the heart is relaxing.
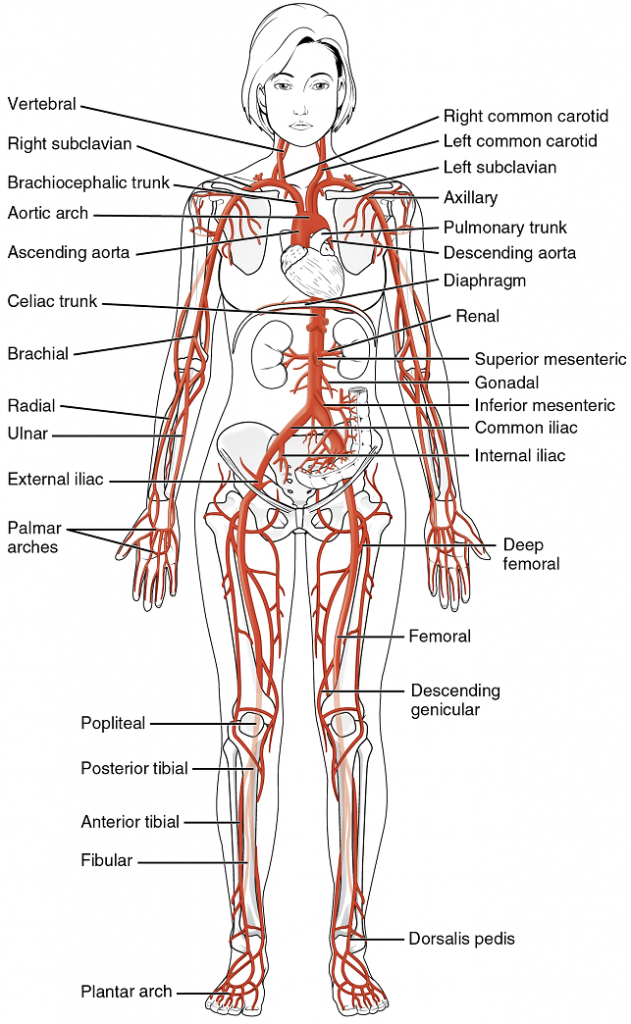
After exiting the heart, the ascending aorta moves in a superior direction for approximately 5 cm and ends at the sternal angle. Following this ascent, it reverses direction, forming a graceful arc to the left, called the aortic arch. The aortic arch descends toward the inferior portions of the body and ends at the level of the intervertebral disk between the fourth and fifth thoracic vertebrae. Beyond this point, the descending aorta continues close to the bodies of the vertebrae and passes through an opening in the diaphragm. Superior to the diaphragm, the aorta is called the thoracic aorta, and inferior to the diaphragm, it is called the abdominal aorta. The abdominal aorta terminates when it bifurcates into the two common iliac arteries at the level of the fourth lumbar vertebra. See Figure 55 for an illustration of the ascending aorta, the aortic arch, and the initial segment of the descending aorta plus major branches.
Coronary Circulation
The first vessels that branch from the ascending aorta are the paired coronary arteries (see Figure 42), which arise from two of the three sinuses in the ascending aorta just superior to the aortic semilunar valve. These sinuses contain the aortic baroreceptors and chemoreceptors critical to maintain cardiac function. The left coronary artery arises from the left posterior aortic sinus. The right coronary artery arises from the anterior aortic sinus. Normally, the right posterior aortic sinus does not give rise to a vessel.
The coronary arteries encircle the heart, forming a ring-like structure that divides into the next level of branches that supplies blood to the heart tissues.
Aortic Arch Branches
There are three major branches of the aortic arch: the brachiocephalic artery, the left common carotid artery, and the left subclavian (literally “under the clavicle”) artery. As you would expect based upon proximity to the heart, each of these vessels is classified as an elastic artery.
The brachiocephalic artery is located only on the right side of the body; there is no corresponding artery on the left. The brachiocephalic artery branches into the right subclavian artery and the right common carotid artery. The left subclavian and left common carotid arteries arise independently from the aortic arch but otherwise follow a similar pattern and distribution to the corresponding arteries on the right side (see Figure 14).
Each subclavian artery supplies blood to the arms, chest, shoulders, back, and central nervous system.
The common carotid artery divides into internal and external carotid arteries. The right common carotid artery arises from the brachiocephalic artery and the left common carotid artery arises directly from the aortic arch. The branches of the carotid arteries supply blood to numerous structures within the head and neck. Each internal carotid artery initially forms an expansion known as the carotid sinus, containing the carotid baroreceptors and chemoreceptors. Like their counterparts in the aortic sinuses, the information provided by these receptors is critical to maintaining cardiovascular homeostasis (see Figure 13).
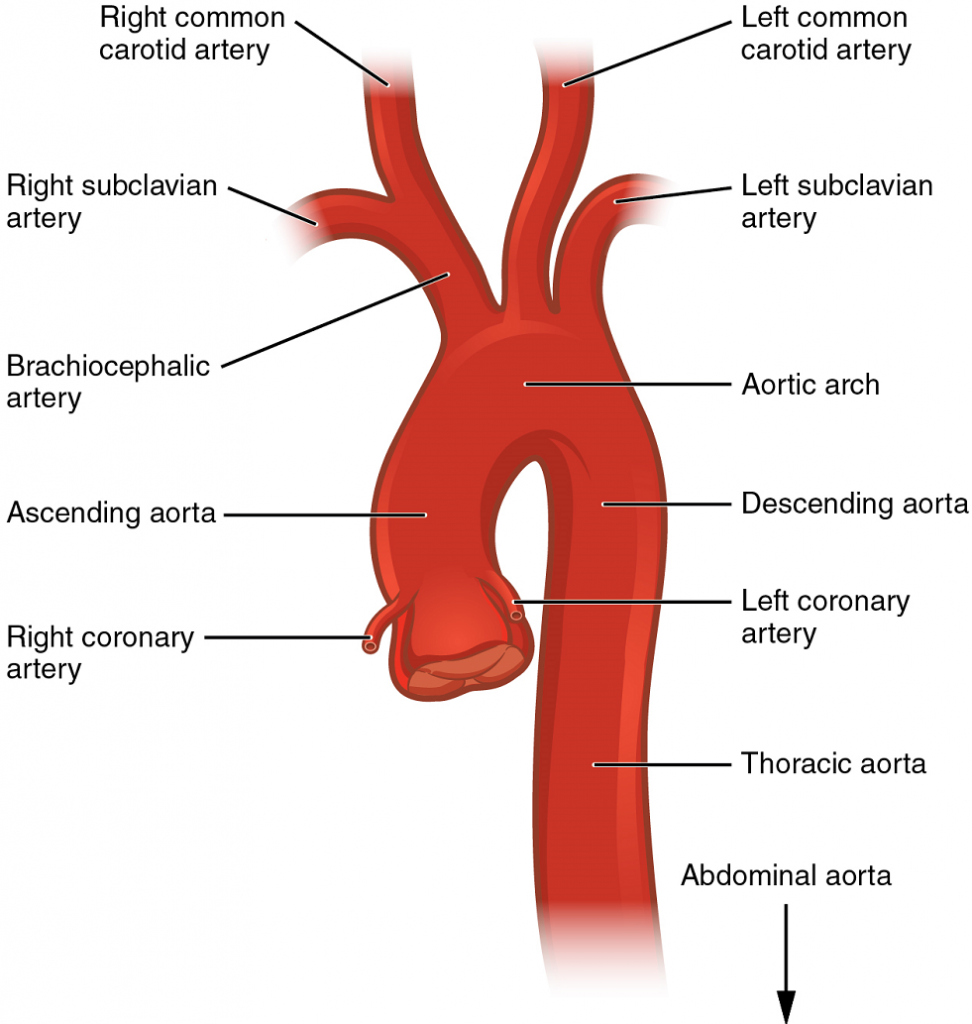
Vessel | Description |
---|---|
Aorta | Largest artery in the body; originates from the left ventricle and descends to the abdominal region then bifurcates into the left and right common iliac arteries at the level of the fourth lumbar vertebra |
Ascending aorta | Initial portion of the aorta; rises superiorly from the left ventricle for a distance of approximately 5 cm |
Aortic arch | Graceful arc to the left that connects the ascending aorta to the descending aorta; ends at the intervertebral disk between the fourth and fifth thoracic vertebrae |
Descending aorta | Continues inferiorly from the end of the aortic arch; subdivided into the thoracic aorta and the abdominal aorta |
Thoracic aorta | Portion of the descending aorta superior to the aortic hiatus |
Abdominal aorta | Portion of the aorta inferior to the aortic hiatus; ends at its bifurcation into the left common iliac artery and the right common iliac artery |
Thoracic Aorta and Major Branches
The thoracic aorta begins at the level of vertebra T5 and continues through to the diaphragm at the level of T12, initially traveling within the mediastinum to the left of the vertebral column. As it passes through the thoracic region, the thoracic aorta gives rise to several branches (Figure 15).
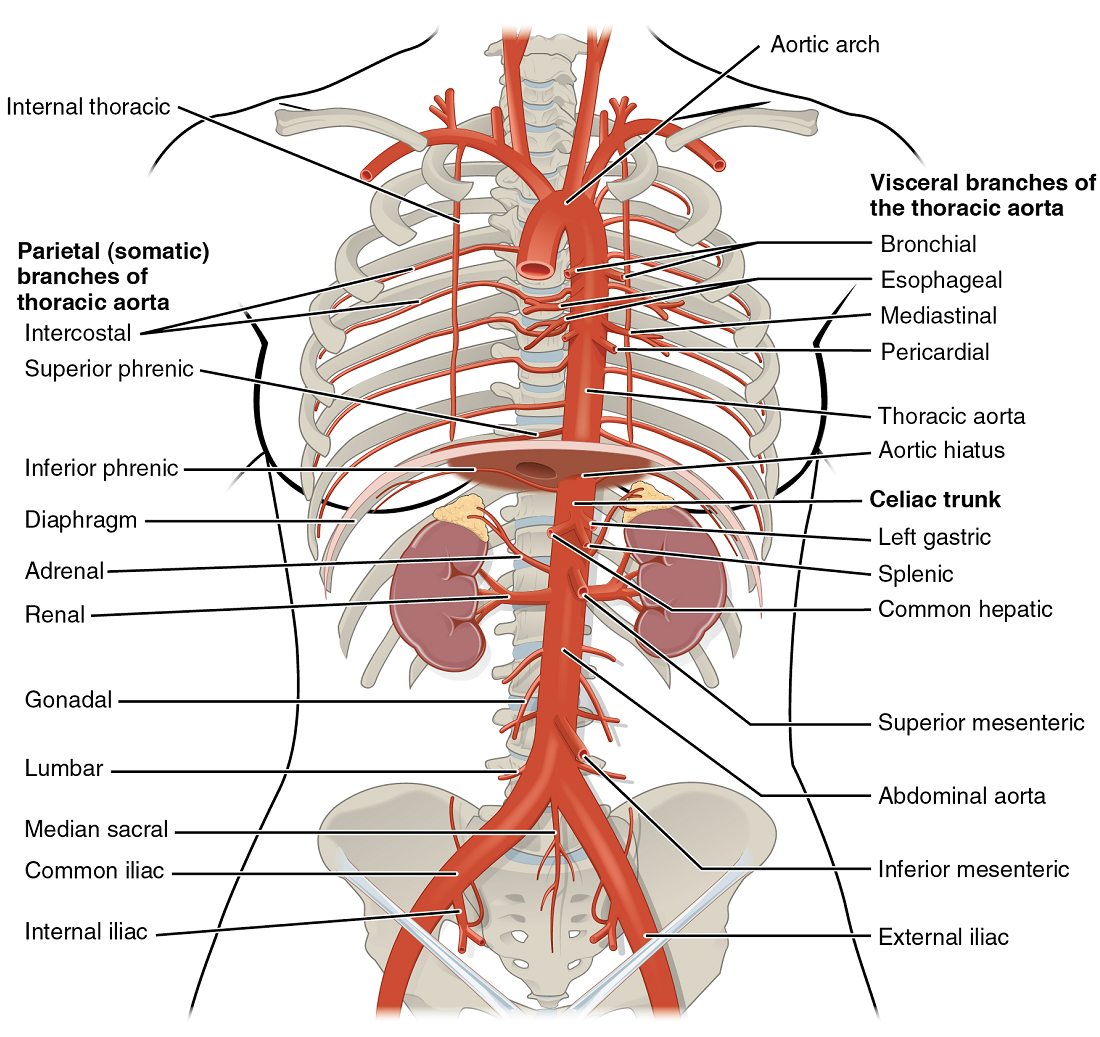
Abdominal Aorta and Major Branches
After crossing through the diaphragm, the thoracic aorta is called the abdominal aorta. This vessel remains to the left of the vertebral column and is embedded in adipose tissue behind the peritoneal cavity. It formally ends at approximately the level of vertebra L4, where it bifurcates to form the two (left and right) common iliac arteries. Before this division, the abdominal aorta gives rise to several important branches. The common iliac arteries provide blood to the pelvic region and ultimately to the lower limbs.
Arteries Serving the Upper and Lower Limbs
Arteries Serving the Upper Limbs: As each subclavian artery exits the thorax into the axillary region, it is renamed the axillary artery. Although each axillary artery does branch and supply blood to the region near the head of the humerus (via the humeral circumflex arteries), the majority of the vessel continues into the upper arm, or brachium, and becomes the brachial artery.
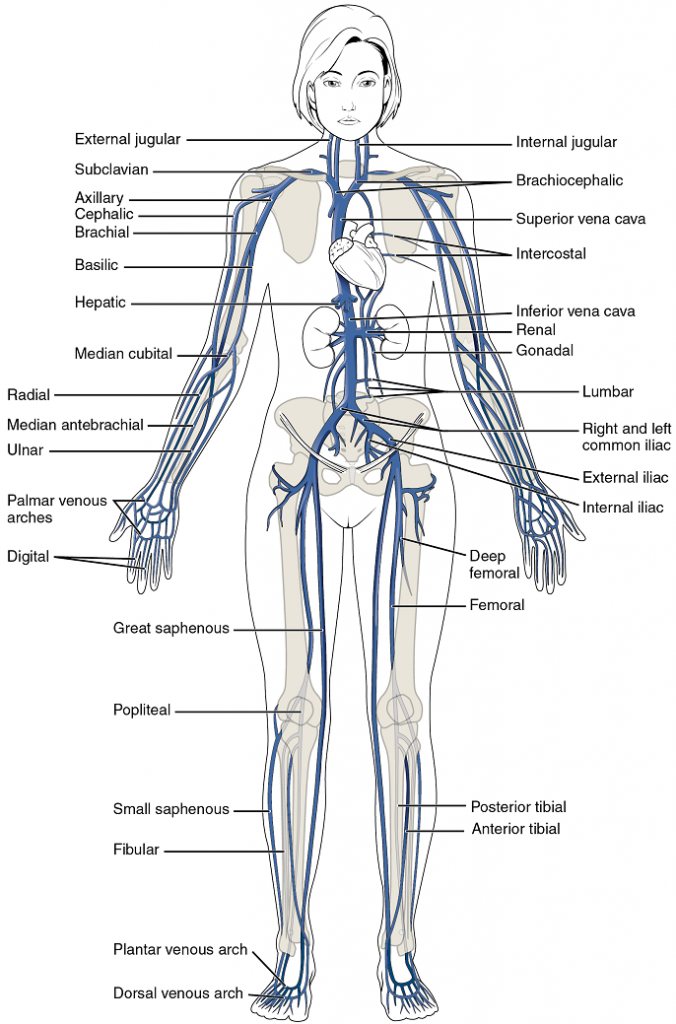
Arteries Serving the Lower Limbs: Each external iliac artery exits the body cavity and enters the femoral region of the lower leg. As it passes through the body wall, it is renamed the femoral artery. Each femoral artery gives rise to the genicular artery, which provides blood to the region of the knee. As each femoral artery passes posterior to the knee near the popliteal fossa, it is called the popliteal artery. Each popliteal artery branches into anterior and posterior tibial arteries.
Overview of Systemic Veins
Systemic veins return blood to the right atrium. Since the blood has already passed through the systemic capillaries, it will be relatively low in oxygen concentration (Figure 16).
The right atrium receives all of the systemic venous return. Most of the blood flows into either the superior vena cava or inferior vena cava. If you draw an imaginary line at the level of the diaphragm, systemic venous circulation from above that line will generally flow into the superior vena cava; this includes blood from the head, neck, chest, shoulders, and upper limbs. The exception to this is that most venous blood flow from the coronary veins flows directly into the coronary sinus and from there directly into the right atrium. Beneath the diaphragm, systemic venous flow enters the inferior vena cava, that is, blood from the abdominal and pelvic regions and the lower limbs.
The Superior and Inferior Vena Cavae
The Superior Vena Cava: The superior vena cava drains most of the body superior to the diaphragm (Figure 17). On both the left and right sides, the subclavian vein forms when the axillary vein passes through the body wall from the axillary region. Each subclavian vein joins with the external and internal jugular veins from the head and neck to form the brachiocephalic vein.
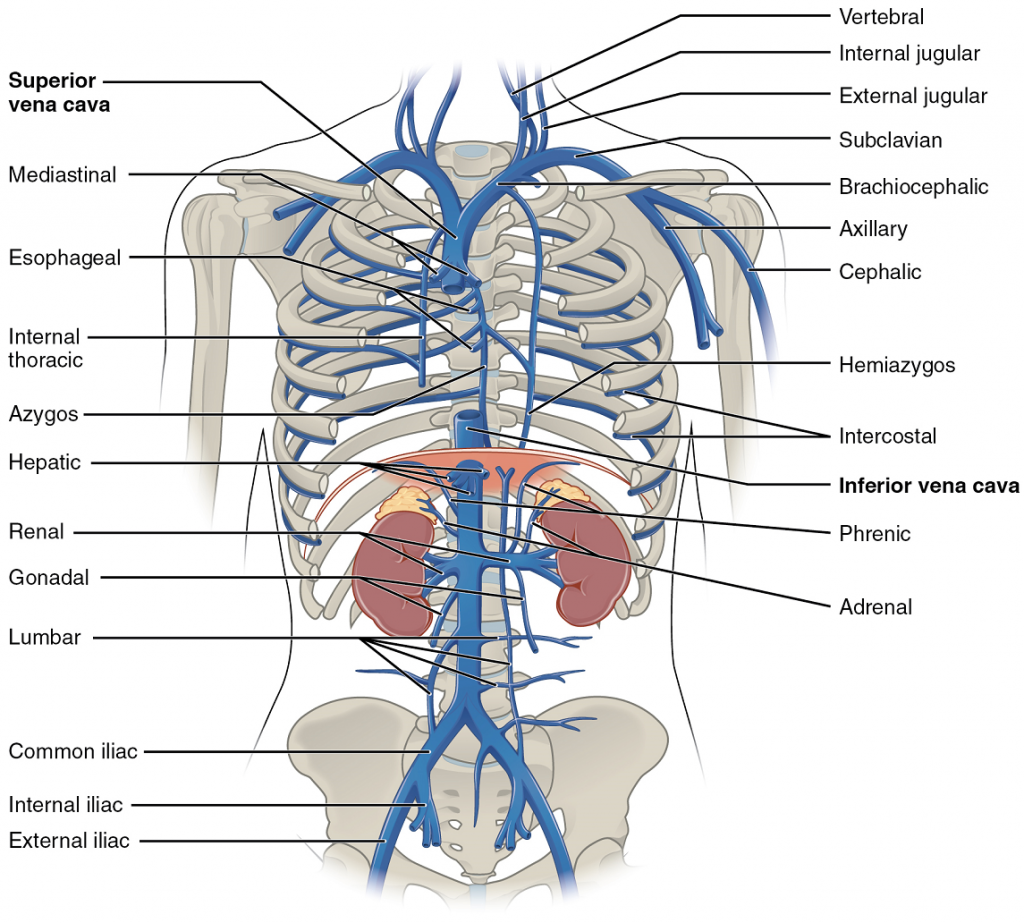
The Inferior Vena Cava: Most of the blood inferior to the diaphragm drains into the inferior vena cava before it is returned to the heart (see Figure 17). Lying just beneath the parietal peritoneum in the abdominal cavity, the inferior vena cava parallels the abdominal aorta, where it can receive blood from abdominal veins.
Veins Draining the Lower Limbs
As each femoral vein penetrates the body wall from the femoral portion of the upper limb, it becomes the external iliac vein, a large vein that drains blood from the leg to the common iliac vein (Figure 18). The pelvic organs and integument drain into the internal iliac vein on either side of the body, which forms from several smaller veins in the region, including the umbilical veins that run on either side of the bladder. The external and internal iliac veins combine near the inferior portion of the sacroiliac joint on either side to form the common iliac vein. In addition to blood supply from the external and internal iliac veins, the middle sacral vein drains the sacral region into the common iliac vein. Similar to the common iliac arteries, the two common iliac veins come together at the level of L5 to form the inferior vena cava.
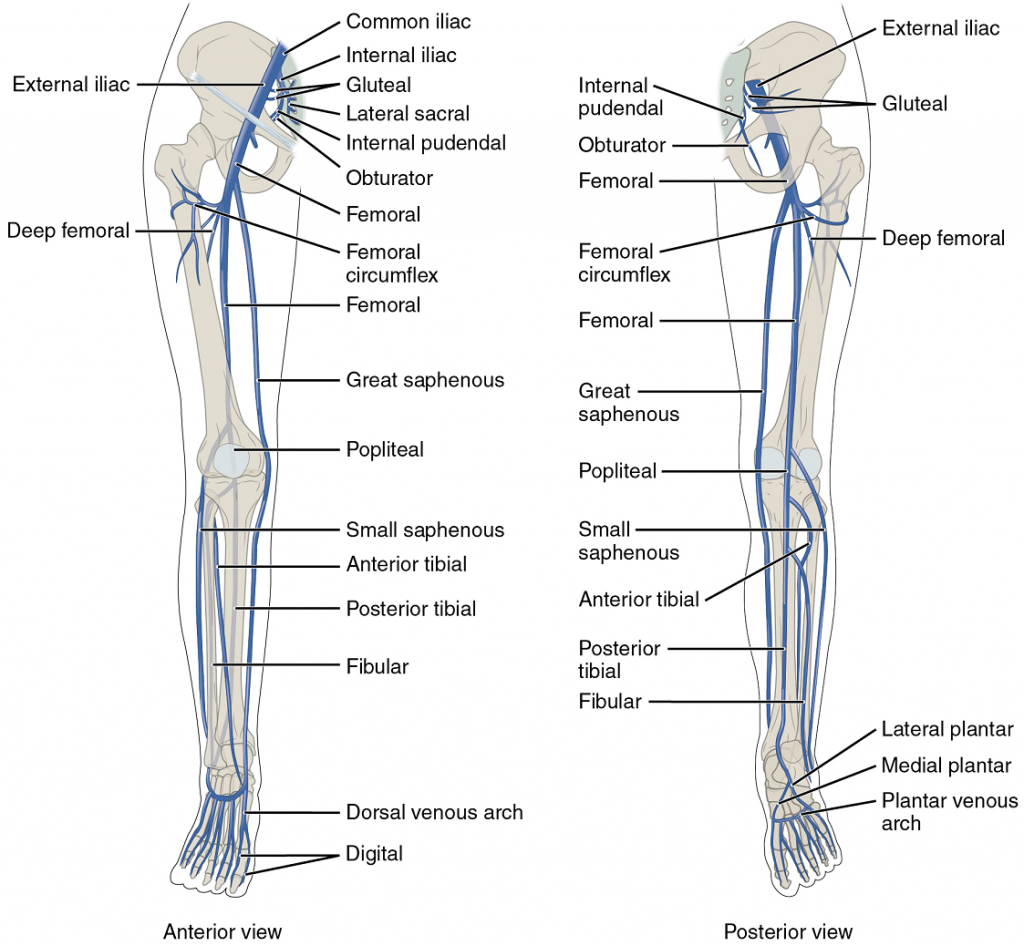
Part 1: Structure and function of blood vessels
Part 2: Capillary Exchange
Part 3: Blood flow, blood pressure, and resistance
Part 4: Hemostatic Regulation of the Vascular System
Part 5: Circulatory Pathways
Blood vessel that conducts blood away from the heart; may be a conducting or distributing vessel.
Very small artery that leads to a capillary.
Smallest of the blood vessels where physical exchange occurs between the blood and tissue cells surrounded by interstitial fluid.
Small vessel leading from the capillaries to veins.
Blood vessel that conducts blood toward the heart.
Parts of the circulatory system involving blood flow to and from almost all the tissues in the body (other than the pulmonary circuit)
Parts of the circulatory system involving blood flow to and from the lungs.
Space inside of a tube, hollow organ or blood vessel.
(Also, tunica interna) innermost lining or tunic of a vessel.
Middle layer or tunic of a vessel (except capillaries).
(Also, tunica adventitia) outermost layer or tunic of a vessel (except capillaries).
The most abundant of three protein fibres found in the extracellular matrix of connective tissues.
Hormones that cause vasoconstriction or release of NO.
A type of loose connective tissue proper that shows little specialization with cells dispersed in the matrix.
Constriction of blood vessels
Dilation (increased internal diameter) of blood vessels.
Delivery of blood through a capillary bed.
Extracellular fluid in the small spaces between cells not contained within blood vessels.
(Also, red blood cell) mature myeloid blood cell that is composed mostly of hemoglobin and functions primarily in the transportation of oxygen and carbon dioxide.
(Also, emigration) process by which leukocytes squeeze through adjacent cells in a blood vessel wall to enter tissues.
(also, white blood cell) colorless, nucleated blood cell, the chief function of which is to protect the body from disease.
Passive diffusion of a substance with the aid of a membrane protein.
Type of capillary with pores or fenestrations in the endothelium that allow for rapid passage of certain small materials.
Rarest type of capillary, which has extremely large intercellular gaps in the basement membrane in addition to clefts and fenestrations; found in areas such as the bone marrow and liver where passage of large molecules occurs.
Import of material into the cell by formation of a membrane-bound vesicle.
Export of a substance out of a cell by formation of a membrane-bound vesicle.
Diffusion of water molecules down their concentration gradient across a selectively permeable membrane.
Larger number recorded when measuring arterial blood pressure; represents the maximum value following ventricular contraction.
Lower number recorded when measuring arterial blood pressure; represents the minimal value corresponding to the pressure that remains during ventricular relaxation.
Average driving force of blood to the tissues; approximated by taking diastolic pressure and adding 1/3 of pulse pressure.
Insufficient blood flow to the tissues.
Lack of oxygen supply to the tissues.
Noises created by turbulent blood flow through the vessels.
Right common carotid artery arises from the brachiocephalic artery, and the left common carotid arises from the aortic arch; gives rise to the external and internal carotid arteries; supplies the respective sides of the head and neck.
continuation of the axillary artery in the brachium; supplies blood to much of the brachial region; gives off several smaller branches that provide blood to the posterior surface of the arm in the region of the elbow; bifurcates into the radial and ulnar arteries at the coronoid fossa
Continuation of the external iliac artery after it passes through the body cavity; divides into several smaller branches, the lateral deep femoral artery, and the genicular artery; becomes the popliteal artery as it passes posterior to the knee.
Continuation of the femoral artery posterior to the knee; branches into the anterior and posterior tibial arteries.
Branch from the popliteal artery that gives rise to the fibular or peroneal artery; supplies blood to the posterior tibial region.
One of the seven bones that make up the posterior foot; includes the calcaneus, talus, navicular, cuboid, medial cuneiform, intermediate cuneiform, and lateral cuneiform bones.
Forms from the anterior tibial artery; branches repeatedly to supply blood to the tarsal and dorsal regions of the foot.
Primary and most potent catecholamine hormone secreted by the adrenal medulla in response to short-term stress; also called adrenaline.
Secondary catecholamine hormone secreted by the adrenal medulla in response to short-term stress; also called noradrenaline.
Collection of hormones secreted by the thyroid gland with wide-ranging metabolic affects.
Branch of the autonomic nervous system associated with resting systems ("rest and digest").
In blood, the liquid extracellular matrix composed mostly of water that circulates the formed elements and dissolved materials throughout the cardiovascular system.
Process of producing red blood cells.
Elevated level of hemoglobin, whether adaptive or pathological.
Deficiency of red blood cells or hemoglobin, often linked to iron deficiency.
(Also, thrombocytes) one of the formed elements of blood that consists of cell fragments broken off from megakaryocytes.
Period of time when the heart muscle is relaxed and the chambers fill with blood.
Lowest (most inferior) part of the brain, controlling many autonomic functions including heart rate, breathing, and digestion.
Tenth cranial nerve; responsible for the autonomic control of organs in the thoracic and upper abdominal cavities.
Gas produced by the blood vessel endothelium that acts as a powerful vasodilator, active over short distances (between nearby cells) for very short times (seconds).Not to be confused with the anesthetic nitrous oxide (N2O).
Small pocket in the ascending aorta near the aortic valve that are the locations of the baroreceptors (stretch receptors) and chemoreceptors that trigger a reflex that aids in the regulation of vascular homeostasis.
Small pocket near the base of the internal carotid arteries that are the locations of the baroreceptors and chemoreceptors that trigger a reflex that aids in the regulation of vascular homeostasis.
Arises from the common carotid artery and begins with the carotid sinus; goes through the carotid canal of the temporal bone to the base of the brain; combines with branches of the vertebral artery forming the arterial circle; supplies blood to the brain.
Change in voltage of a cell membrane in response to a stimulus that results in transmission of an electrical signal; unique to neurons and muscle fibres.
Branch of the autonomic nervous system associated with emergency systems ("fight of flight").
Sensory receptor (stretch receptor) sensitive to changes in pressure.
Sensory receptor that senses chemical concentrations.
(Also, vasopressin) hypothalamic hormone that is stored by the posterior pituitary and that signals the kidneys to reabsorb water.
Molecule (usually a protein) that catalyzes chemical reactions.
Located at the juncture of the distal convoluted tubule and the afferent and efferent arterioles of the glomerulus; plays a role in the regulation of renal blood flow and glomerular filtration rate.
Hormone produced and secreted by the adrenal cortex that stimulates sodium and fluid retention and increases blood volume and blood pressure.
Region of the diencephalon inferior to the thalamus that functions in neural and endocrine signaling.
Glycoprotein that triggers the bone marrow to produce RBCs; secreted by the kidney in response to low oxygen levels.
A ring-shaped smooth muscle that can open or close a passage in the body.
Similar to hormones, lipids produced by various cells (not glands), usually at the site of an injury or other issue, that are active over a short distance (targeting other cells in the same tissue).
(Also, immunoglobulin) antigen-specific protein secreted by plasma cells.
Physiological process by which bleeding ceases.
Right subclavian arises from the brachiocephalic artery, whereas the left subclavian artery arises from the aortic arch; gives rise to the internal thoracic, vertebral, and thyrocervical arteries; supplies blood to the arms, chest, shoulders, back, and central nervous system.
Continuation of the subclavian artery as it penetrates the body wall and enters the axillary region; supplies blood to the region near the head of the humerus (humeral circumflex arteries); the majority of the vessel continues into the brachium and becomes the brachial artery.
Area inferior to the shoulder joint (armpit, or underarm).
Large systemic vein that returns blood to the heart from the superior portion of the body.
Large systemic vein that returns blood to the heart from the inferior portion of the body.
Large, thin-walled vein on the posterior surface of the heart that lies within the atrioventricular sulcus and drains the heart myocardium directly into the right atrium.
The single large vessel exiting the right ventricle that divides to form the right and left pulmonary arteries.
Valves located at the base of the pulmonary trunk and at the base of the aorta.
Single large vessel exiting the right ventricle that divides to form the right and left pulmonary arteries.
Small, grape-like sac that performs gas exchange in the lungs (pl.= alveoli).
Two sets of paired vessels, one pair on each side, that are formed from the small venules leading away from the pulmonary capillaries that flow into the left atrium.
Largest artery in the body, originating from the left ventricle and descending to the abdominal region where it bifurcates into the common iliac arteries at the level of the fourth lumbar vertebra; arteries originating from the aorta distribute blood to virtually all tissues of the body.
Portion of the aorta that continues downward past the end of the aortic arch; subdivided into the thoracic aorta and the abdominal aorta.
The direction towards the head.
Arc that connects the ascending aorta to the descending aorta; ends at the intervertebral disk between the fourth and fifth thoracic vertebrae.
Portion of the aorta that continues downward past the end of the aortic arch; subdivided into the thoracic aorta and the abdominal aorta.
Portion of the descending aorta superior to the aortic hiatus.
Portion of the aorta inferior to the aortic hiatus and superior to the common iliac arteries.
Branches of the ascending aorta that supply blood to the heart; the left coronary artery feeds the left side of the heart, the left atrium and ventricle, and the interventricular septum; the right coronary artery feeds the right atrium, portions of both ventricles, and the heart conduction system.
Single vessel located on the right side of the body; the first vessel branching from the aortic arch; gives rise to the right subclavian artery and the right common carotid artery; supplies blood to the head, neck, upper limb, and wall of the thoracic region.
A central compartment in the thoracic cavity located intermediate to the left and right pleural cavities.
Branch of the aorta that leads to the internal and external iliac arteries.
Continuation of the external iliac artery after it passes through the body cavity; divides into several smaller branches, the lateral deep femoral artery, and the genicular artery; becomes the popliteal artery as it passes posterior to the knee.
(Left and right) located deep in the thoracic cavity; becomes the axillary vein as it enters the axillary region; drains the axillary and smaller local veins near the scapular region; leads to the brachiocephalic vein.
Major vein in the axillary region; drains the upper limb and becomes the subclavian vein.
One of a pair of veins that form from a fusion of the external and internal jugular veins and the subclavian vein; subclavian, external and internal jugulars, vertebral, and internal thoracic veins lead to it; drains the upper thoracic region and flows into the superior vena cava.
Drains the upper leg; receives blood from the great saphenous vein, the deep femoral vein, and the femoral circumflex vein; becomes the external iliac vein when it crosses the body wall.
One of a pair of veins that flows into the inferior vena cava at the level of L5; the left common iliac vein drains the sacral region; divides into external and internal iliac veins near the inferior portion of the sacroiliac joint.