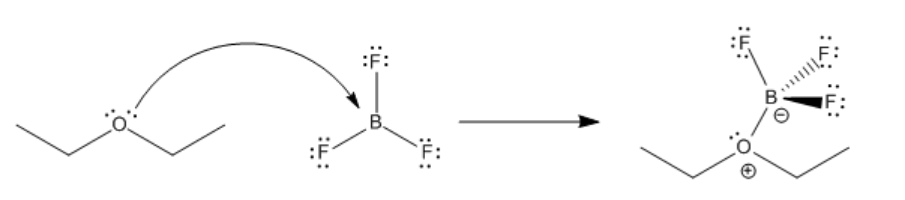
Figure 2.4.1 – Formation of a Lewis acid-base complex.
An example of a coordination complex is hexaaquo cobalt dichloride, Co(H2O)6Cl2. This compound contains a Co2+ ion. This electrophilic metal ion is coordinated by six nucleophilic water ligands. Because the water molecules are neutral, the complex still has a 2+ charge overall. There are two chloride counterions to balance the charge, but the chlorides are not attached to the complex ion.
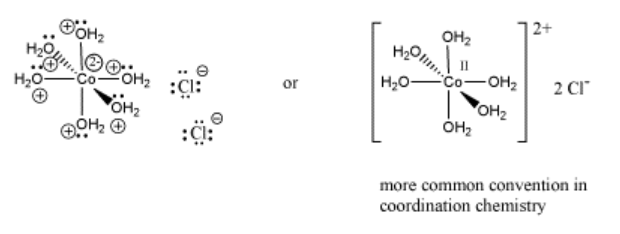
Notice that the usual Lewis conventions are usually abandoned in drawing coordination complexes of transition metals. With so many different nucleophiles sticking to the Lewis acid, the number of formal charges that must be drawn becomes very cumbersome. Usually the oxidation state of the metal cation is denoted. The oxidation state essentially means the charge on the metal cation and it is written in Roman numerals beside the metal atom. In addition, the overall charge on the Lewis acid-base complex is given, with square brackets indicating that the charge belongs to the entire complex within the brackets. Exactly where that charge resides is up to the reader to consider.
Coordination complexes are frequently useful in mining and metallurgy. For example, nickel can be extracted from nickel ore by converting the nickel into Ni(CO)4 via addition of carbon monoxide. Ni(CO)4, or tetracarbonyl nickel, is a gas that can be easily separated from the solid ore. When removed from the presence of carbon monoxide, the coordination complex decomposes back into Ni and CO.
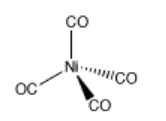
Exercise 2.4.1
Problem 1. These questions concern the formation of tetracarbonyl nickel.
a) Based on what you know about transition metals, is the nickel most likely a Lewis acid or a Lewis base?
b) Draw the Lewis structure for carbon monoxide, CO. Make sure each atom has an octet. Make sure you add formal charges.
c) Based on formal charge considerations, which atom in carbon monoxide will bind to the nickel?
d) Using arrow notation, show the coordination of CO to Ni. Do this one step at a time, showng each CO molecule binding to the nickel and the new complex that results after each step.
e) Why does nickel bind four carbon monoxide ligands, and not three or five?
- Answer a):
- Lewis acid
- Answer b):
- Answer c):
- Carbon will be nucleophilic due to the lone pair electrons and the negative charge.
- Answer d):
- Answer e):
- Ni0 is d10 and with 4 CO ligands the nickel tetracarbonyl complex has 18 electrons.
Another example is seen in the liquid extraction of metals from ores. Often, a metal is coordinated by some kind of ligand that changes the solubility of the metal atom, so that it can be extracted from the ore with a solvent. The solvent is usually water, but other liquids could be used. One of the easiest cases to picture on paper is the leaching of gold from gold ores with cyanide.
Often, coordination of a ligand to a metal changes many properties of the metal, in addition to changing its solubility. Transition metals have different oxidation states, meaning they can give up different numbers of electrons and become cations with different charges. Sometimes, this event happens more easily upon coordination. In the case of gold, the gold atom can react with air and become a gold cation in the presence of cyanide.
Cyanide is an anion, so it would be added as a salt, such as sodium cyanide, NaCN, or potassium cyanide, KCN. The resulting complex, Au(CN)2, is actually a complex anion, and it would be associated with one of the counterions added, such as KAu(CN)2. It is not completely clear why the gold binds two cyanides, but binding two ligands is common in the chemistry of gold and silver, and is based on molecular orbital considerations that are beyond the level of this course.

This complex salt is water-soluble, so it can be removed from the ore with water. Later, it must be converted back into pure gold via electrochemical reactions. These reactions are not related to acid-base chemistry and will not be covered in this course.
Exercise 2.4.2
Problem 2 These questions concern the formation of the potassium dicyano gold complex.
a) Based on what you know about transition metals, is the gold most likely a Lewis acid or a Lewis base?
b) Draw the Lewis structure for cyanide, CN–. Make sure each atom has an octet. Make sure you add formal charges.
c) Based on formal charge considerations, which atom in cyanide will bind to the gold?
d) Using arrow notation, show the coordination of cyanide to Au+. (We will simplify and assume the gold has already been oxidized). Do this one step at a time, showing each cyanide molecule binding to the gold ion and the new complex that results after each step.
- Answer a):
- Lewis acid
- Answer b):
- Answer c):
- Carbon will be nucleophilic due to the lone pair electrons and the negative charge.
- Answer d):
Perhaps some of the most interesting coordination complexes involve transition metals in biology. The most familiar of these compounds is hemoglobin. Hemoglobin is a complex protein that contains a crucial iron atom. The iron is present as a 2+ cation, but the heme ring that binds to the iron has a 2- charge, so overall there is no charge on the complex. The iron is still electrophilic, however, and it can bind an oxygen molecule. Much more oxygen can be carried in the bloodstream this way than if the oxygen simply dissolved in the blood.
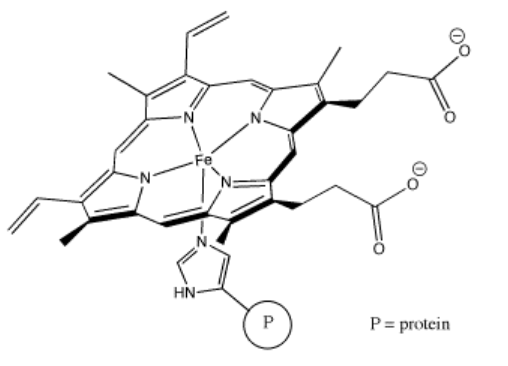
Exercise 2.4.3
Problem 3. These questions concern the formation of the oxyhemoglobin.
a) Based on what you know about transition metals, is the iron most likely a Lewis acid or a Lewis base?
b) Draw the Lewis structure for oxygen molecule, O2. Make sure each atom has an octet. Make sure you see if there are any formal charges.
N.B. This Lewis structure may not agree completely with the molecular orbital view of the molecule, but it is all we have to work with for our current method of understanding coordination.
c) Using arrow notation, show the coordination of oxygen to Fe2+.
d) There has been a great deal of research into the exact geometry of the dioxygen complex. Based on your Lewis structure of oxygen, show how you think the oxygen is attached to the iron. How would you describe the geometry?
- Answer a):
- Lewis acid
- Answer b):
- Answer c):
- Answer d):
- The bound oxygen would be predicted to have a trigonal planar electronic (bend molecular) geometry based on the Lewis structure.
There is another interesting event that happens when oxygen binds to the iron in hemoglobin. After binding O2, the iron actually transfers a single electron to the oxygen, becoming a Fe3+ cation. Although deoxyhemoglobin, the Fe2+ or Fe(II) species, is purple, many Fe3+ or Fe(III) compounds are red. Thus, oxyhemoglobin is red.
It is also interesting to note that we are used to seeing iron-containing materials turn a sort of red-brown color when they have been exposed to oxygen for a long time, especially in the presence of water and salts. Our cars, trains, and bridges eventually rust like the iron in their steel turns to reddish iron oxide. William Tolman, a researcher in bioinorganic chemistry at the University of Minnesota, likes to raise the following question: we rely on iron complexes to carry oxygen through our salt-water bloodstream, so why don’t we get rusty?
The answer is that, in a sense, we do. It has been estimated that after several passes through our bloodstream, a hemoglobin molecule meets its end when the oxygen fails to detach and the iron complex decomposes to iron oxide. That’s one reason why you need to eat a diet that contains iron, to replenish your iron stores in order to make new hemoglobin on a regular basis.
To see some additional problems that will help you get to know coordination compounds, go to the main coordination compound chapter. In particular, it is really useful to be able to count electrons in coordination chemistry. Counting electrons is just like learning Lewis structures, except that instead of going to a maximum count of eight electrons, we can go to a maximum of eighteen electrons. That’s because the noble gas configuration for a transition metal would have eighteen electrons, not eight. To learn how to count electrons in coordination complexes, go here.
Note
A general introduction to coordination compounds is given in the context of Lewis acids and bases on another page.
Much of reactivity can be understood in Lewis acidity terms. Perhaps the simplest examples of reactions are the formation of Lewis acid-base complexes. In a Lewis acid-base complex, a Lewis base has simply shared a pair of electrons with a Lewis acid, forming a new bond.
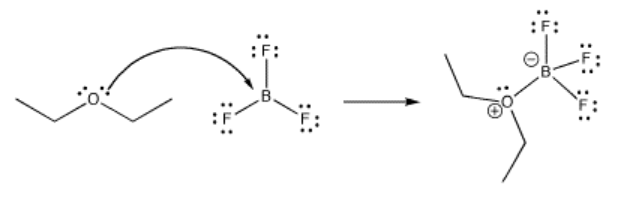
Frequently, metal atoms or ions act as Lewis acids. They can often accept electrons from a number of different Lewis bases at once, forming “complexes” or “complex ions” (“complex” meaning they are formed from individual parts that connect together). These Lewis bases (also called “ligands”) are said to be “coordinated” to the metal, meaning they are stuck to the metal via the electron pair that they share with it.
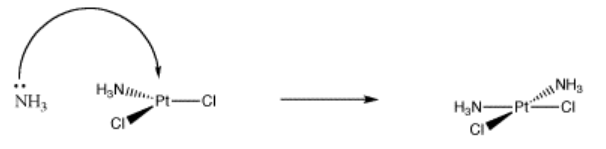
A number of examples of ligands (compounds with lone pairs that can bind to metals) are shown in the following table.
Table 2.4.1 – Some Common Ligands.
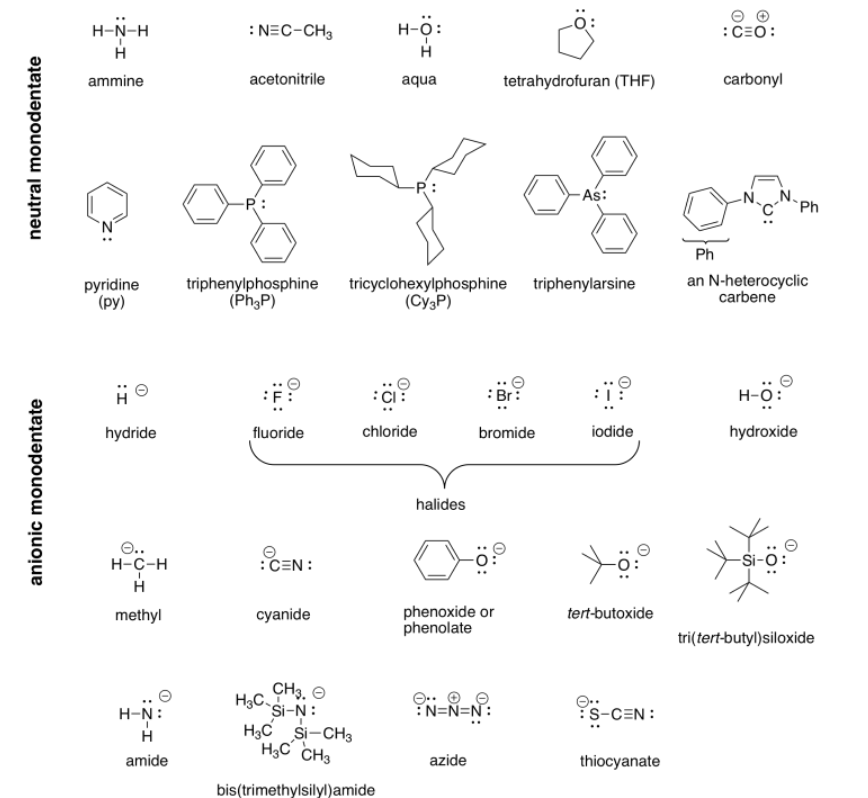
“Coordination complexes” play important roles in biology as well as economically important processes. Probably the most familiar coordination complex in biology is hemoglobin. It can coordinate with an additional dioxygen molecule and carry the oxygen through the bloodstream, delivering oxygen to tissues much more efficiently.
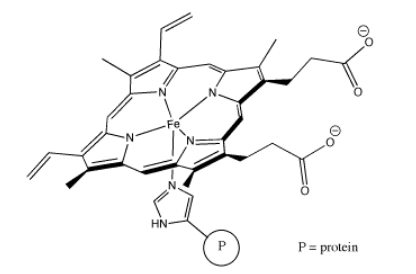
A very common coordination complex in industrial use is Wilkinson’s catalyst, (PPh3)3RhCl. Wilkinson’s catalyst is used to make a number of transformations more efficient; most notably, it is used in hydrogenation reactions. Chemical transformations of this sort are commonly used in making pharmaceuticals and other high-demand materials.
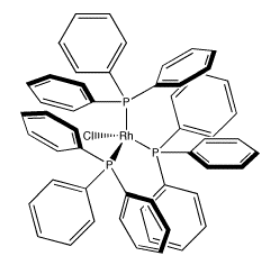
Because coordination compounds can sometimes be anions or cations, there is a convention used to tell the reader which part of the formula is connected together, and which part is the counterion(s). The part listed in square brackets consists of ligands bonding to a central metal; the part outside the brackets is the counterion(s). For example, [(H2O)6Co]Cl2 consists of a Co2+ ion bound to six water molecules. Two separate chloride anions are found nearby.
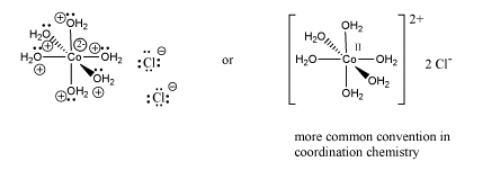
Exercise 2.4.4
Draw structures for the following coordination compounds.
- K2[PtCl6]
- K3[Fe(CN)6]
- [(NH3)4CoCl2]Cl (two isomers)
- [(NH3)3CoCl3] (two isomers)
- [(NH3)2Ag]PF6 (it’s Ag+; that’s a single PF6– counterion)
- Na[HgCl3]
- Cd(NH3)4Cl2 (two isomers)
- Li2[CoCl4]
- K[Rh(CO)2I2]
- [Cu(NH3)4](SO4)2
- Na2[Ni(CN)4]
- [Fe(OH2)6](NO3)2
- K3[Fe(SCN)6]
- K2[Zn(OH)4]
- Pt(NH3)2Cl2 (two isomers)
- [Ru(NH3)5Cl]Cl2
- Li[Sn(OH)3]
- K4[Mn(CN)6]
- Ni(CO)4
- [Co(NH3)5NO2](NO2)2 (two isomers based on how the ligand connects to the metal)
- [Co(NH3)5SCN]Cl2 (two isomers possible)
- [VO]SO4 (challenge: why might you draw the V-O bond differently than the other metal-ligand bonds so far?)
- [VO2]PF6
- Na2[HgS2]
- K[MnO4]
- Exercise 2.4.5
Problem CC1.2.
Identify the geometries of each of the complexes in Problem CC1.1.
Exercise 2.4.6
Note:
Some of the complexes in Problem CC1.1. have interesting stories. For example, problem (a) was reported many years ago by Alexander Shilov to be capable of breaking C-H bonds. This reaction has the potential to convert methane, routinely burned off in petroleum fields because it is a safety hazard, into useful products. All of that methane is currently being converted directly into greenhouse gases, and that’s a real problem. (Astronauts have reported that, at night on Earth, the petroleum fields of the Middle East shine brighter than major cities.) This reaction has recently received intensive study in the lab of John Bercaw at Caltech.
Problem (i) is an economically important catalyst, used in the production of acetic acid in the Monsanto Process, originally developed by BASF but improved upon by Monsanto. These days, it is being supplanted by the same complex with iridium instead of rhodium; that complex drives the more environmentally-friendly Cativa Process. The new process was developed by BP. Acetic acid is a sort of heavy-duty worker molecule used in the production of paints, plastics, pharmaceuticals and other commodities.
Problem (p) is a member of a class of potent anti-cancer drugs that are particularly effective against testicular and ovarian tumours. Exactly how they worked was the subject of study for many years, and was finally deduced by Steve Lippard (MIT) and Amy Rosenzweig (Northwestern).
Problem (t) is used in the Mond Process for the purification of nickel ores. We use nickel principally in making steel, but also in other alloys.
Vanadyl salts such as (w) are sometimes found in minerals such as cavansite, giving them an intense blue color.