Maintenance of the Body
Unit 4: The Respiratory System
Unit outline
Part 1: Anatomy of the Respiratory System
- Conducting zone
- Respiratory zone
- The Lungs
Part 2: The Process of Breathing – Pulmonary Ventilation
- Pulmonary ventilation
- Nervous control of ventilation
- Factors that affect the rate and depth of ventilation
- Respiratory volumes and capacities
Part 3: Gas Exchange
- External respiration
- Internal respiration
Part 4: Transport of Gases
- Oxygen transport in blood
- Factors affecting oxygen dissociation from hemoglobin
- Carbon dioxide transport in the blood
Part 5: Modifications in Respiratory Functions
- Hyperventilation
- Hypoxia
Learning Objectives
At the end of this unit, you should be able to:
I. Describe the location and function(s) of the major components of the human respiratory system.
II. Explain the mechanism of ventilation (inspiration and expiration) in humans, including the roles of the structures involved.
III. Describe the nervous control of breathing.
IV. Describe how carbon dioxide, oxygen and hydrogen ions control the rate of breathing.
V. Interpret a spirogram and define the respiratory volumes and capacities a spirogram depicts.
VI. Explain the basic principle governing the reciprocal exchange of gases between the alveoli and the blood, and between the blood and individual cells.
VII. Describe the mechanisms by which oxygen and carbon dioxide are transported in the blood.
VIII. Define hyperventilation and specify some of its causes and physiological consequences.
IX. Define hypoxia and specify some of its causes and physiological consequences.
Learning Objectives and Guiding Questions
At the end of this unit, you should be able to complete all the following tasks, including answering the guiding questions associated with each task.
I. Describe the location and function(s) of the major components of the human respiratory system.
- Draw a series of fully-annotated diagrams showing the structure of the respiratory system. Include the location, general structure and function of all the following components:
- External nares
- Nasal cavity
- Internal nares
- Oral cavity
- Pharynx
- Epiglottis
- Glottis
- Larynx
- Trachea
- Bronchus
- Bronchiole
- Alveolus
- Visceral pleura
- Parietal pleura
- Pleural cavity
II. Explain the mechanism of ventilation (inspiration and expiration) in humans, including the roles of the structures involved.
- Describe the mechanism of pulmonary ventilation in humans, including a detailed description of the processes of quiet inspiration (inhalation) and quiet expiration (exhalation). Refer in your explanation to:
- The skeletal muscles required for quiet inhalation
- The two passive processes required for quiet exhalation
- Changes in the volume of the thoracic cavity at each step
- Changes in the intra-alveolar pressure at each step
- The direction of air travel at each step
III. Describe the nervous control of breathing.
- Describe how breathing is modulated by the nervous system, making reference to all of the following structures:
- Diaphragm
- External intercostals
- Internal intercostals
- Pons
- Medulla oblongata
- Pontine respiratory group
- Apneustic center
- Pneumotaxic center
- Ventral respiratory group
- Dorsal respiratory group
- Cerebral cortex
- Peripheral chemoreceptors
- Central chemoreceptors
IV. Describe how carbon dioxide, oxygen and hydrogen ions control the rate of breathing.
- Describe the mechanisms by which each of the following influence the rate of breathing:
- Partial pressure (or concentration) of carbon dioxide gas in the blood
- Partial pressure (or concentration of oxygen gas in the blood
- Concentration of hydrogen ions (protons) in the blood
V. Interpret a spirogram and define the respiratory volumes and capacities a spirogram depicts.
- What are spirograms used for?
- Sketch a spirogram showing several normal breaths followed by one deep inspiration and one forced exhalation.
- Identify the following measurements on a spirogram, and describe what each measurement represents physiologically:
- Tidal volume
- Vital capacity
- Residual volume
- Can “dead space volume” be measured from a spirogram? Briefly justify your answer.
VI. Explain the basic principle governing the reciprocal exchange of gases between the alveoli and the blood, and between the blood and individual cells.
- Define and clearly distinguish oxygenated and deoxygenated blood.
- In the systemic circulation, which type of blood vessels normally contain ‘deoxygenated blood’?
- In the systemic circulation, which type of blood vessels normally contain ‘oxygenated blood’?
- In the pulmonary circulation, name the blood vessels which normally contain ‘deoxygenated blood’.
- In the pulmonary circulation, name the blood vessels which normally contain ‘oxygenated blood’?
- By what transport mechanism do oxygen (O2) and carbon dioxide (CO2) gas move across plasma membranes? Explain why this particular transport mechanism is used by oxygen and carbon dioxide gas.
- What condition drives movement of gases in a certain direction during gas exchange?
- What is the difference in the driving force that promotes pulmonary ventilation compared to the driving force which promotes gas exchange?
- Explain why significant gas exchange in the lungs can only occur in the alveoli, and not in the bronchi or bronchioles.
VII. Describe the mechanisms by which oxygen and carbon dioxide are transported in the blood.
- Describe the two mechanisms by which oxygen gas is transported within the blood, and the relative quantities transported by each mechanism.
- Describe the three mechanisms by which carbon dioxide is transported within the blood, and the relative quantities transported by each mechanism.
- Specify the components of blood that contain:
- Dissolved oxygen gas
- Dissolved carbon dioxide gas
- Oxygen molecules bound to hemoglobin
- Carbon dioxide bound to hemoglobin
- Bicarbonate ions
- For each of the following, describe how it influences the amount of oxygen bound to hemoglobin, and describe how it relates to the ability of hemoglobin to pick up or release oxygen at appropriate locations in the human body:
- Blood pH
- Partial pressure (or concentration) of carbon dioxide in blood
- Blood temperature
VIII. Define hyperventilation and specify some of its causes and physiological consequences.
- Clearly and precisely define “hyperventilation”.
- Describe and explain the effects of hyperventilation on:
- Blood oxygen content
- Blood carbon dioxide content
- Blood pH
- Blood pressure
- Brain (neuron) function
- State the two major causes of hyperventilation and provide at least two specific examples of conditions that could underlie each.
IX. Define hypoxia and specify some of its causes and physiological consequences.
- Clearly and precisely define “hypoxia”.
- For each of the following provide a specific example of a condition or circumstance that would cause it and explain how it causes “hypoxia”.
- A deficiency in atmospheric oxygen
- Ventilatory deficiency
- Diffusion deficiency in the lungs
- Deficiency of hemoglobin (anemic hypoxia)
- Circulatory deficiency (ischemic hypoxia)
- Edema
- State three physiological consequences of hypoxia.
The Respiratory System: Hold your breath. Really! See how long you can hold your breath as you continue reading…How long can you do it? Chances are you are feeling uncomfortable already. A typical human cannot survive without breathing for more than 3 minutes, and even if you wanted to hold your breath longer, your autonomic nervous system would take control. This is because most cells in the body run the oxidative stages of cellular respiration, the process by which energy is produced in the form of adenosine triphosphate (ATP). For oxidative phosphorylation to occur, oxygen (O2) is used as a reactant and carbon dioxide (CO2) is released as a waste product. You may be surprised to learn that although oxygen is a critical need for cells, it is actually the accumulation of carbon dioxide that primarily drives your need to breathe. Carbon dioxide is exhaled and oxygen is inhaled through the respiratory system, which involves muscles to move air into and out of the lungs, passageways through which air moves, and microscopic gas exchange surfaces covered by capillaries. The circulatory system transports gases from the lungs to tissues throughout the body and vice versa.
Part 1: Anatomy of the Respiratory System
The major organs of the respiratory system (Figure 1) function primarily to provide oxygen to body tissues for cellular respiration, remove the waste product carbon dioxide, and help to maintain acid-base balance. Functionally, the respiratory system can be divided into a conducting zone and a respiratory zone. The conducting zone of the respiratory system includes the organs and structures not directly involved in gas exchange. The gas exchange occurs in the respiratory zone.
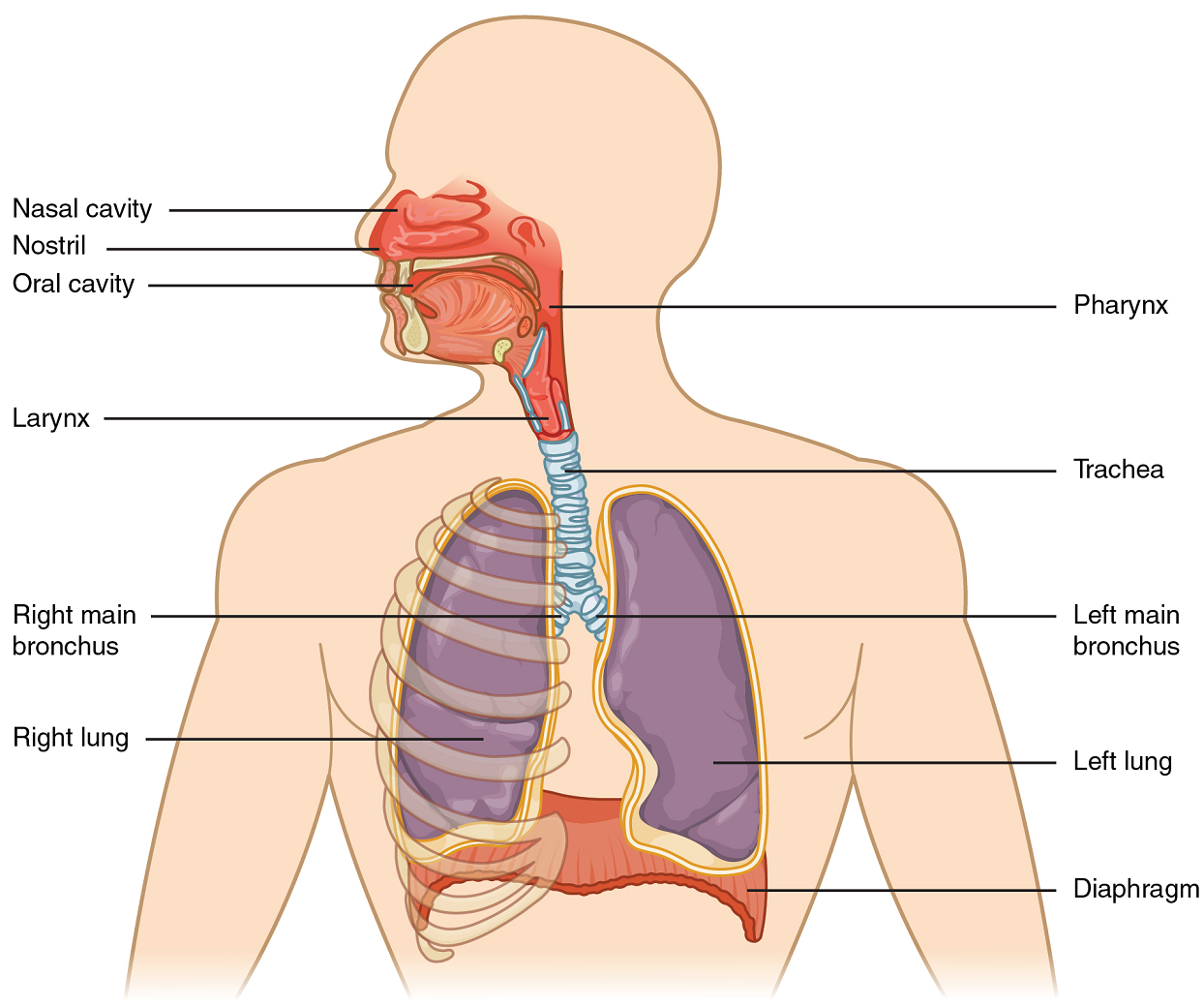
Conducting Zone: The major functions of the conducting zone are to provide a route for incoming and outgoing air, remove debris and pathogens from the incoming air, and warm and humidify the incoming air. In addition, the epithelium of the nasal passages, is essential to sensing odors
The Nose and its Adjacent Structures: The major entrance and exit for the respiratory system is through the nose; some air may also enter through the oral cavity. Large particles, such as dirt, are removed from air by hairs as it enters the external nares (nostrils) of the nose and flows into the nasal cavity (which is separated into left and right sections by the nasal septum). Each lateral wall of the nasal cavity has three conchae (Figure 2) or bony projections, which serve to increase the surface area of the nasal cavity and slow the flow of air in the nose to allow it to be cleaned, warmed and moistened. The nasal cavity is lined by respiratory epithelium (ciliated columnar epithelium) and goblet cells (Figure 3). The goblet cells produce mucus to trap debris and the cilia of the respiratory epithelium beat to clear the mucus from the nasal cavity to the throat to be swallowed. For further protection, goblet cells secrete antibacterial substances. Deep in the nasal cavity, an olfactory epithelium is used to detect odors (as described in the special sense unit of BIOL 1103/1109). Air exits the nasal cavities via the internal nares and moves into the pharynx.
Pharynx: The pharynx is a tube formed by skeletal muscle and lined by mucous membrane that is continuous with the epithelium of the nasal cavities. The pharynx is divided into three major regions: the nasopharynx, the oropharynx, and the laryngopharynx (Figure 3).
The nasopharynx is flanked by anteriorly by the conchae of the nasal cavity, and it serves only as an airway. The oropharynx and laryngopharynx are passageways for both air and food. At the inferior end of the laryngopharynx, the digestive and respiratory systems diverge. Anteriorly, the laryngopharynx opens into the larynx, whereas posteriorly, it enters the esophagus.
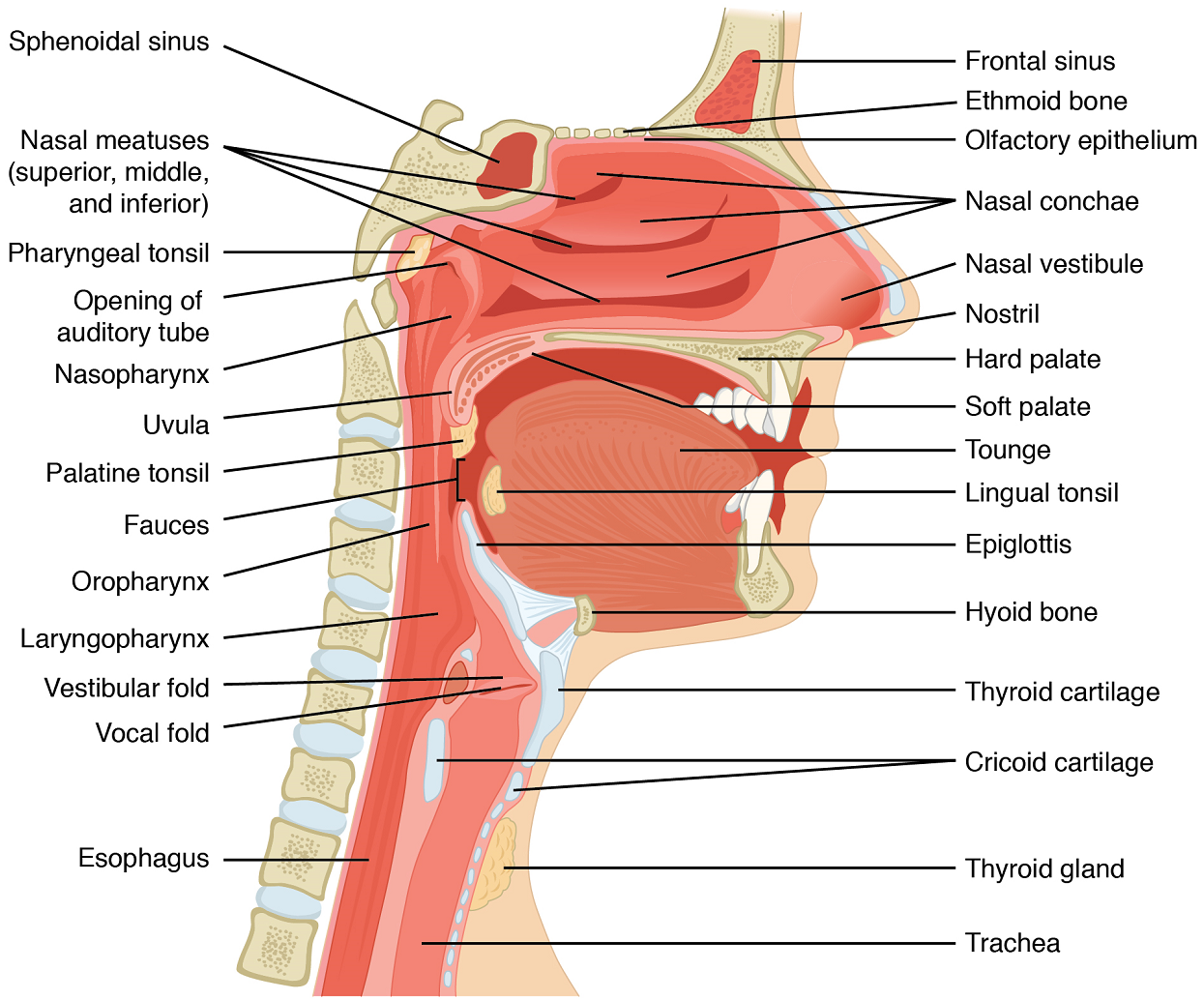
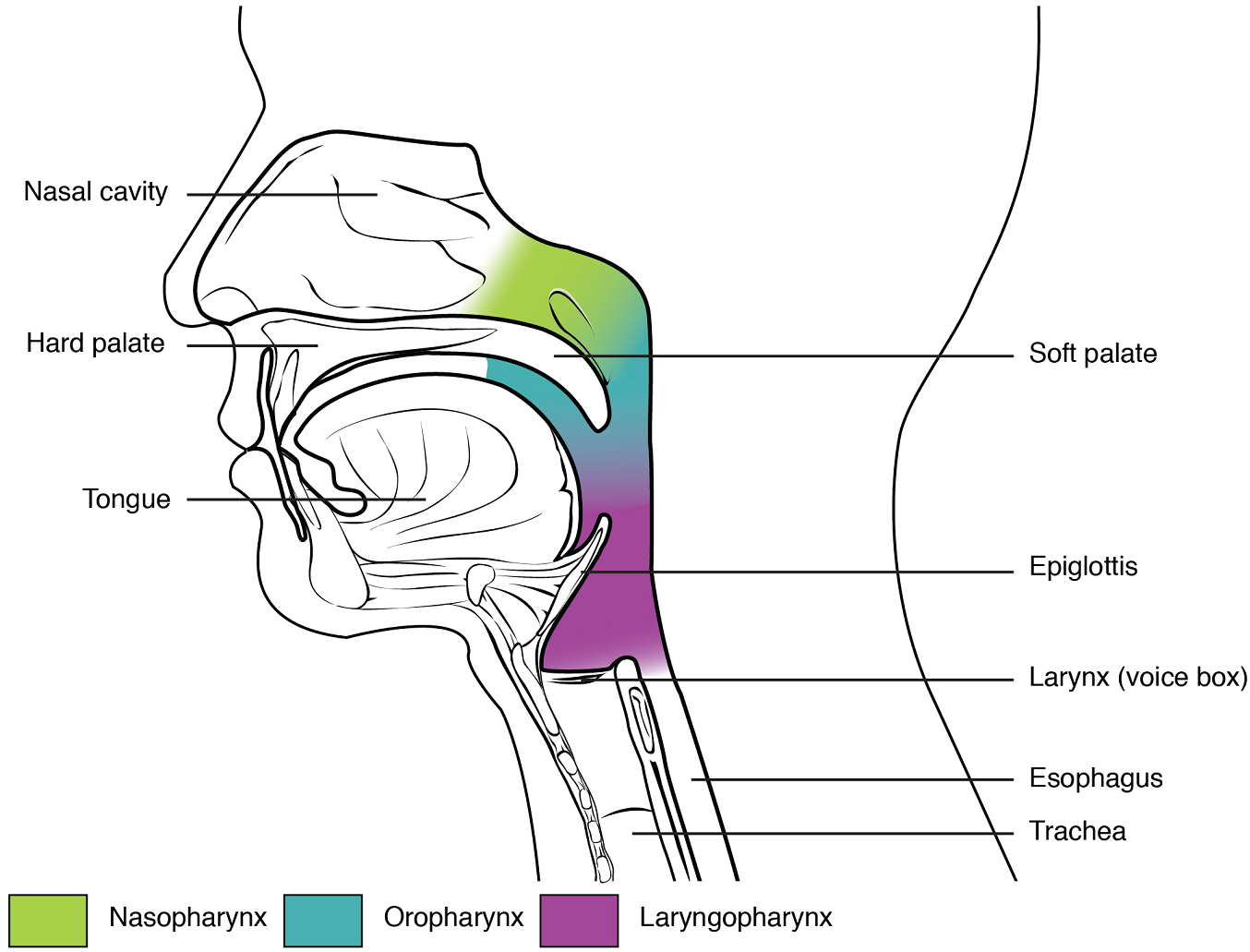
Larynx: The larynx is a cartilaginous structure inferior to the laryngopharynx that connects the pharynx to the trachea and helps regulate the volume of air that enters and leaves the lungs. The structure of the larynx is formed by several pieces of cartilage (Figure 4). Three large cartilage pieces—the thyroid cartilage (anterior; which contains the Adam’s apple or laryngeal prominence), epiglottis (superior), and cricoid cartilage (inferior)—form the major structure of the larynx. Other smaller, paired pieces of cartilage help move the vocal cords for speech.
The epiglottis is a very flexible piece of elastic cartilage that covers the opening of the trachea (see Figure 2). When in the “closed” position, during swallowing, the unattached end of the epiglottis rests on the glottis preventing food and beverages from entering the trachea (as will be described in the digestive system section). The glottis is composed of the vestibular folds, the true vocal cords, and the space between these folds (Figure 5).
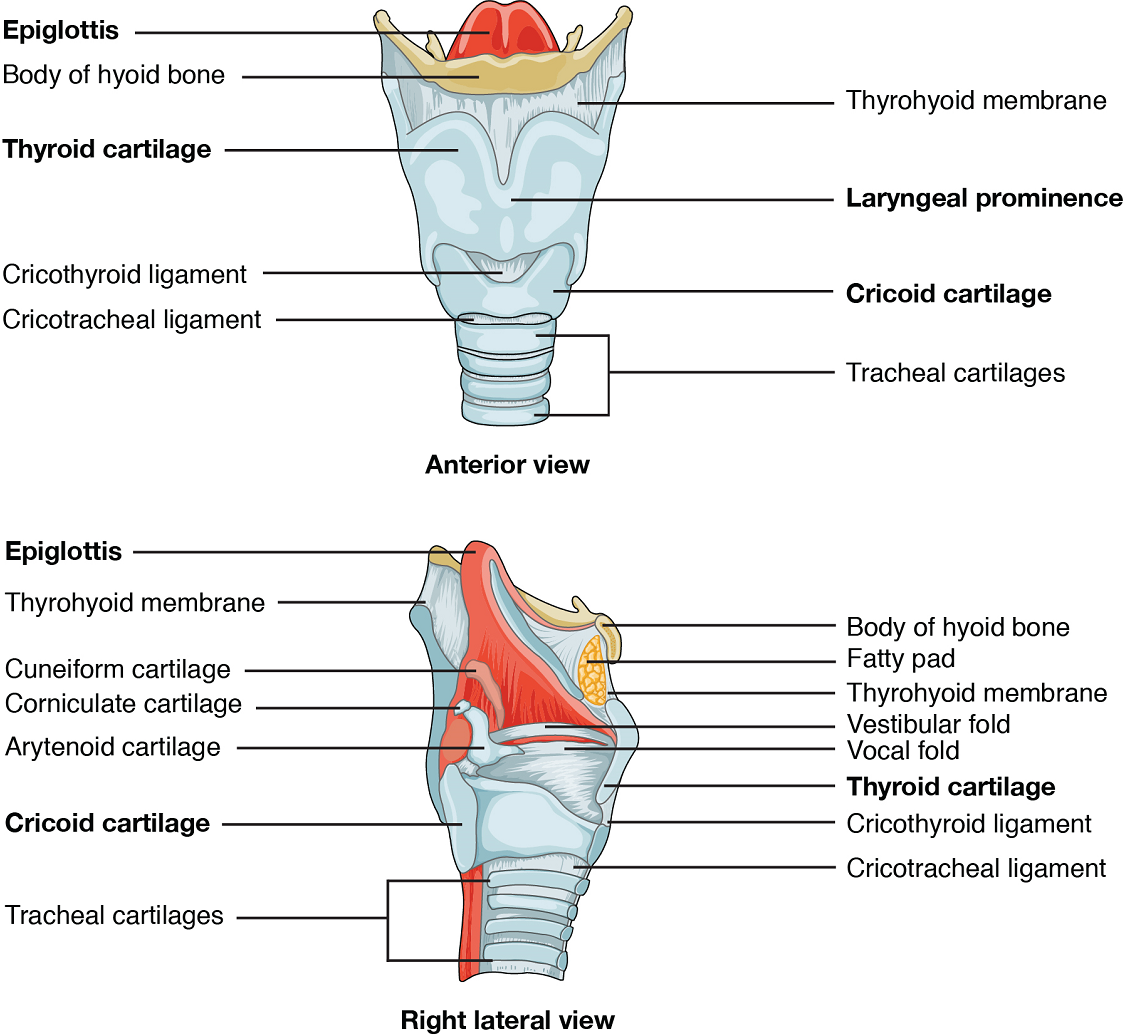
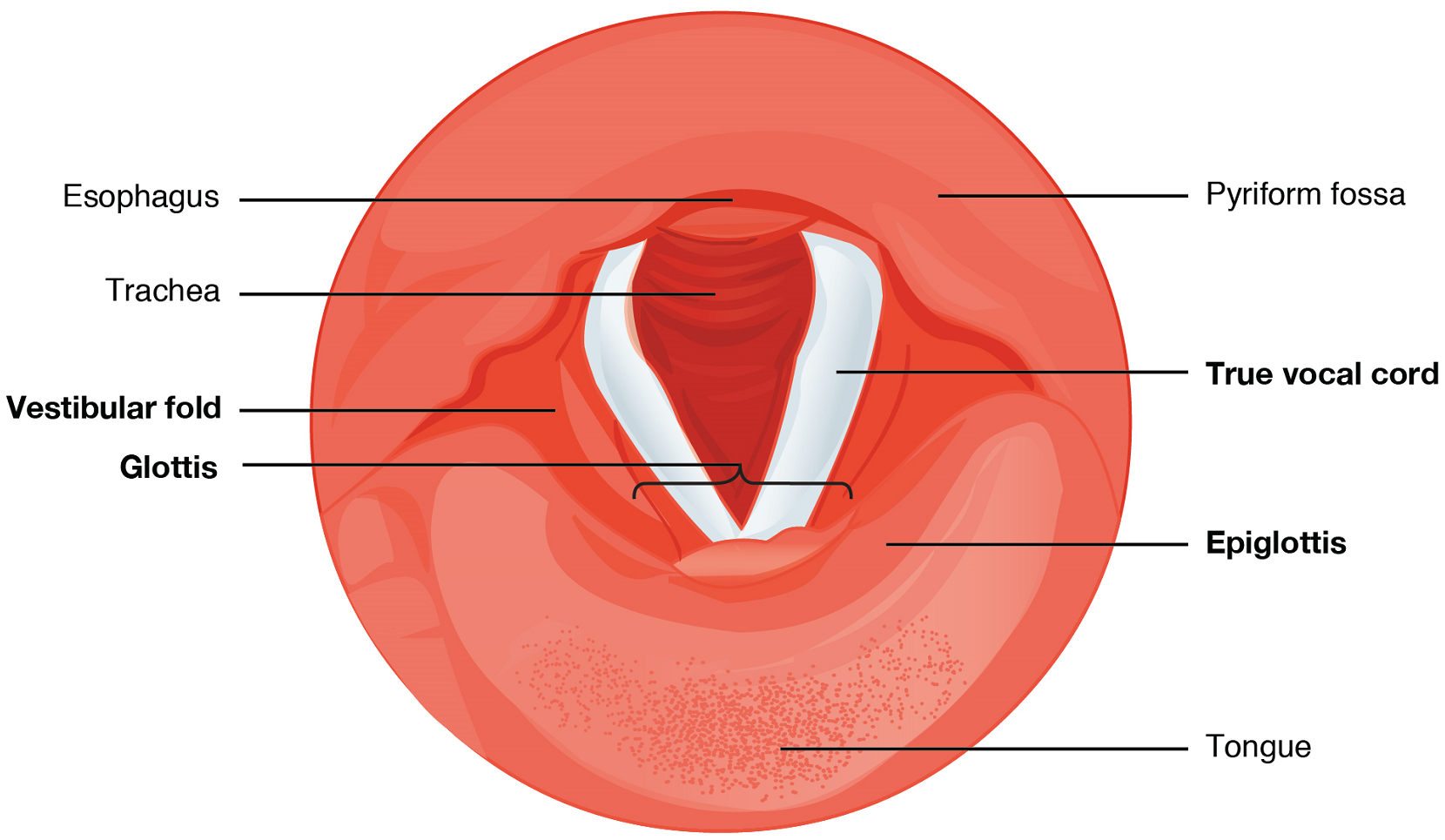
Trachea: The trachea (windpipe) extends from the larynx toward the lungs (Figure 6a) and is formed by 16 to 20 stacked, C-shaped pieces of hyaline cartilage connected by dense connective tissue. The rings of cartilage provide structural support and prevent the trachea from collapsing.
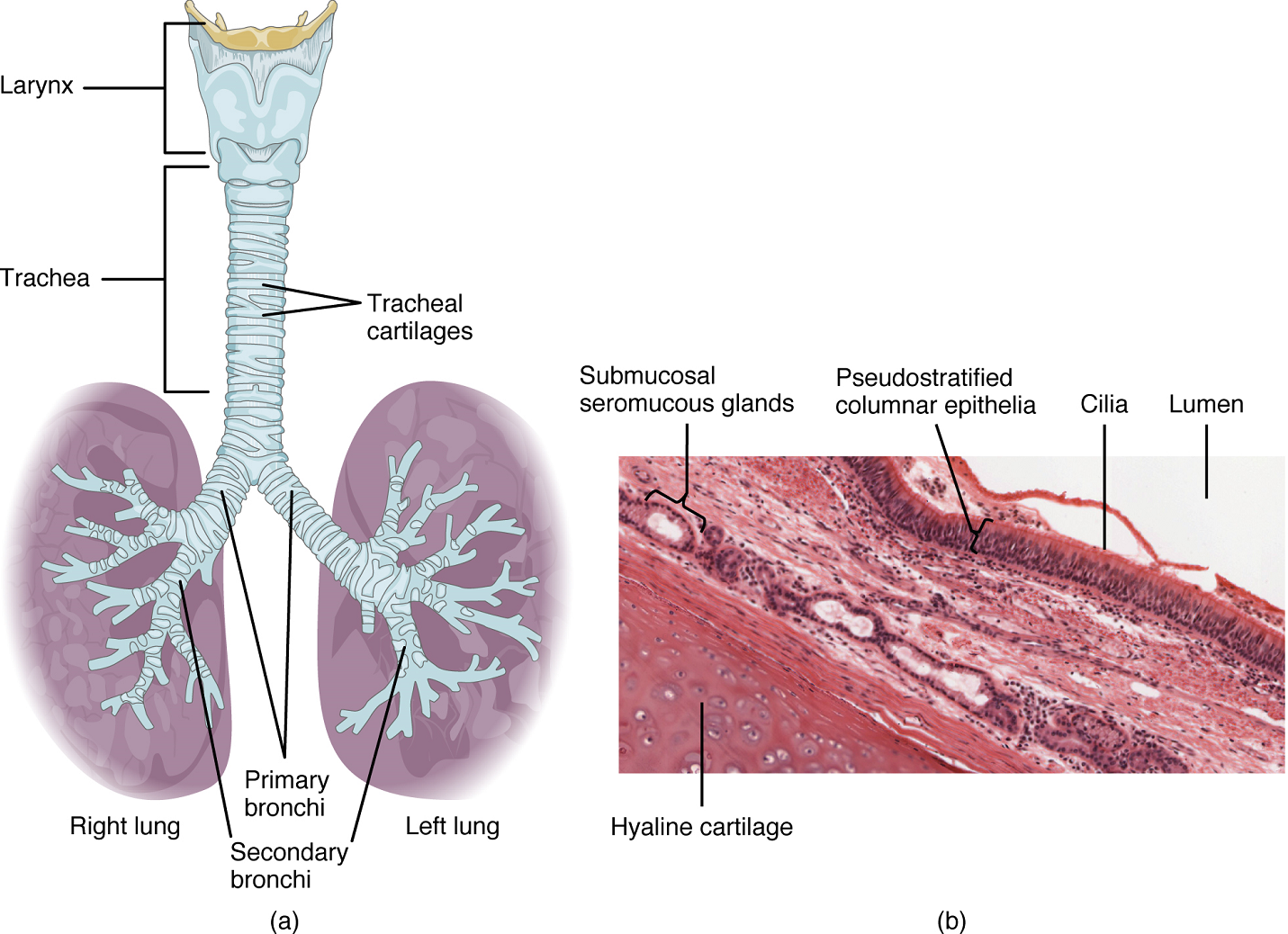
Bronchial Tree: The trachea branches into the right and left primary bronchi (Figure 6b). Rings of cartilage, similar to those of the trachea, support the structure of the bronchi and prevent their collapse. The primary bronchi enter the lungs and each primary bronchus branches into a secondary bronchus and then into a tertiary bronchus and so on. These multiple-branched bronchi are referred to as the bronchial tree. The main function of the bronchi, like other conducting zone structures, is to provide a passageway for air to move into and out of each lung. In addition, the mucous membrane traps debris and pathogens.
Bronchioles branch from the tertiary bronchi. Bronchioles, which are about 1 mm in diameter, further branch until they become the tiny terminal bronchioles, which lead to the structures of gas exchange (Figure 7). There are more than 1000 terminal bronchioles in each lung. The muscular walls of the bronchioles do not contain cartilage like those of the bronchi. This muscular wall can change the size of the tubing to increase or decrease airflow through the tube, for example during exercise.
Respiratory Zone: In contrast to the conducting zone, the respiratory zone includes structures that are directly involved in gas exchange of carbon dioxide and oxygen. The respiratory zone begins where the terminal bronchioles join a respiratory bronchiole, the smallest type of bronchiole (Figure 7), which then leads to an alveolar duct, opening into a cluster of alveoli (called an alveolar sac).
Alveoli: An alveolus is one of the many small, grape-like sacs in an alveolar sac responsible for gas exchange. An alveolus is approximately 200 μm in diameter with elastic walls that allow the alveolus to stretch during air intake, which greatly increases the surface area available for gas exchange. Alveoli are connected to their neighbors by alveolar pores, which help maintain equal air pressure throughout the alveoli and lung (Figure 8).
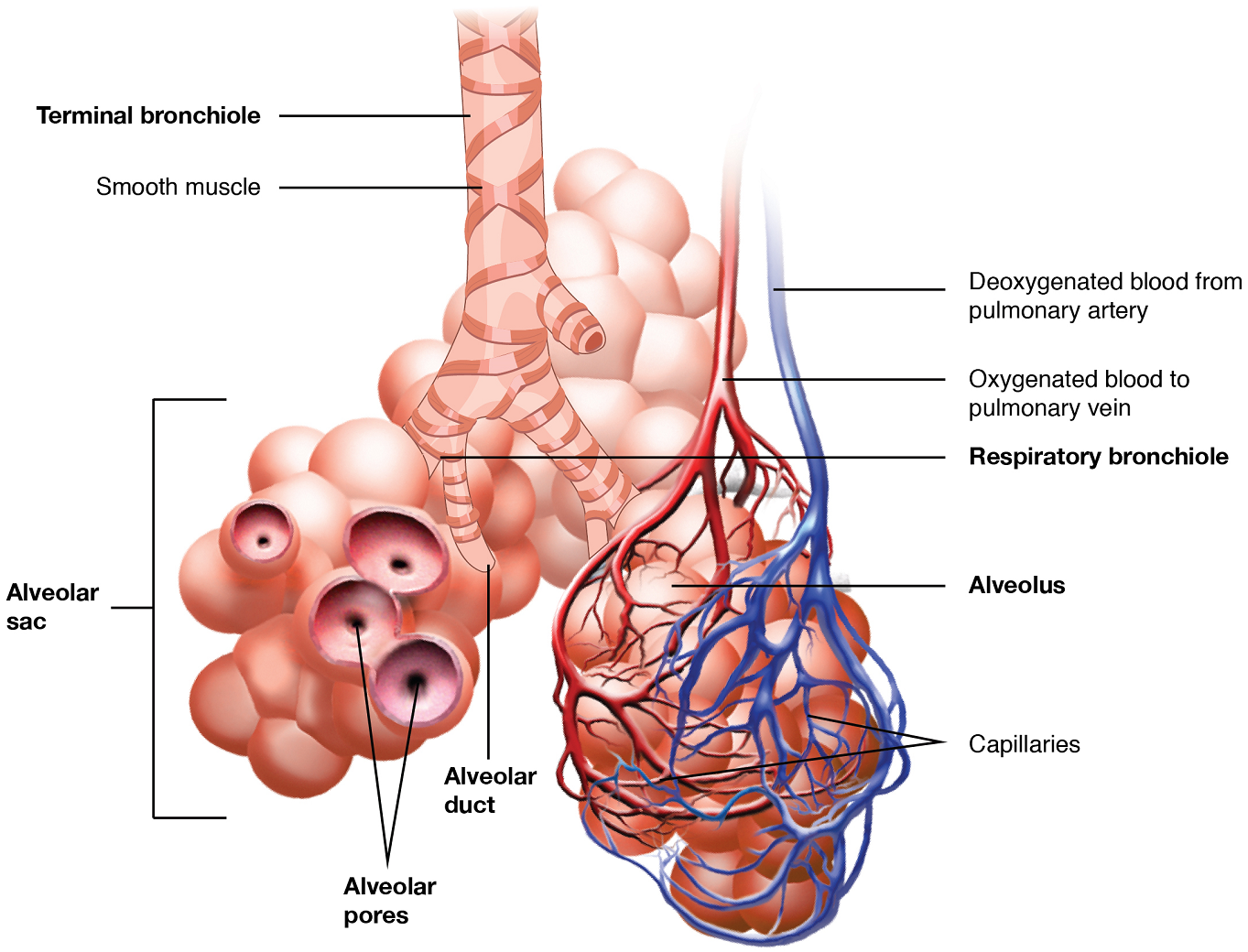
The alveolar wall consists mostly of simple squamous epithelial cells. These cells are about 25 nm thick and are highly permeable to gases.

The simple squamous epithelium formed by type I alveolar cells is attached to a thin, elastic basement membrane. This epithelium is extremely thin and borders the endothelial membrane of capillaries, which is also composed of simple squamous epithelium. Taken together, the alveoli and capillary membranes form a respiratory membrane that is approximately 0.5 mm thick. The respiratory membrane allows gases to cross by simple diffusion, allowing oxygen to be picked up by the blood for transport and carbon dioxide to be released into the air of the alveoli. The respiratory membrane covers a surface area—about 70 square meters.
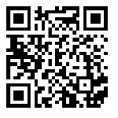
The Lungs: The major organs of the respiratory system; each lung houses structures of both the conducting and respiratory zones starting at the primary bronchi and ending at the alveoli.
Gross Anatomy of the Lungs: The lungs are pyramid-shaped, paired organs that are connected to the trachea by the right and left bronchi; on the inferior surface, the lungs are bordered by the diaphragm. The diaphragm is the flat, dome-shaped muscle located at the base of the lungs and thoracic cavity. The lungs are enclosed by the pleurae. The right lung is shorter and wider than the left lung, and the left lung occupies a smaller volume than the right to allow space for the heart (Figure 9). Each lung is composed of smaller units called lobes. The right lung consists of three lobes: the superior, middle, and inferior lobes. The left lung consists of two lobes: the superior and inferior lobes.
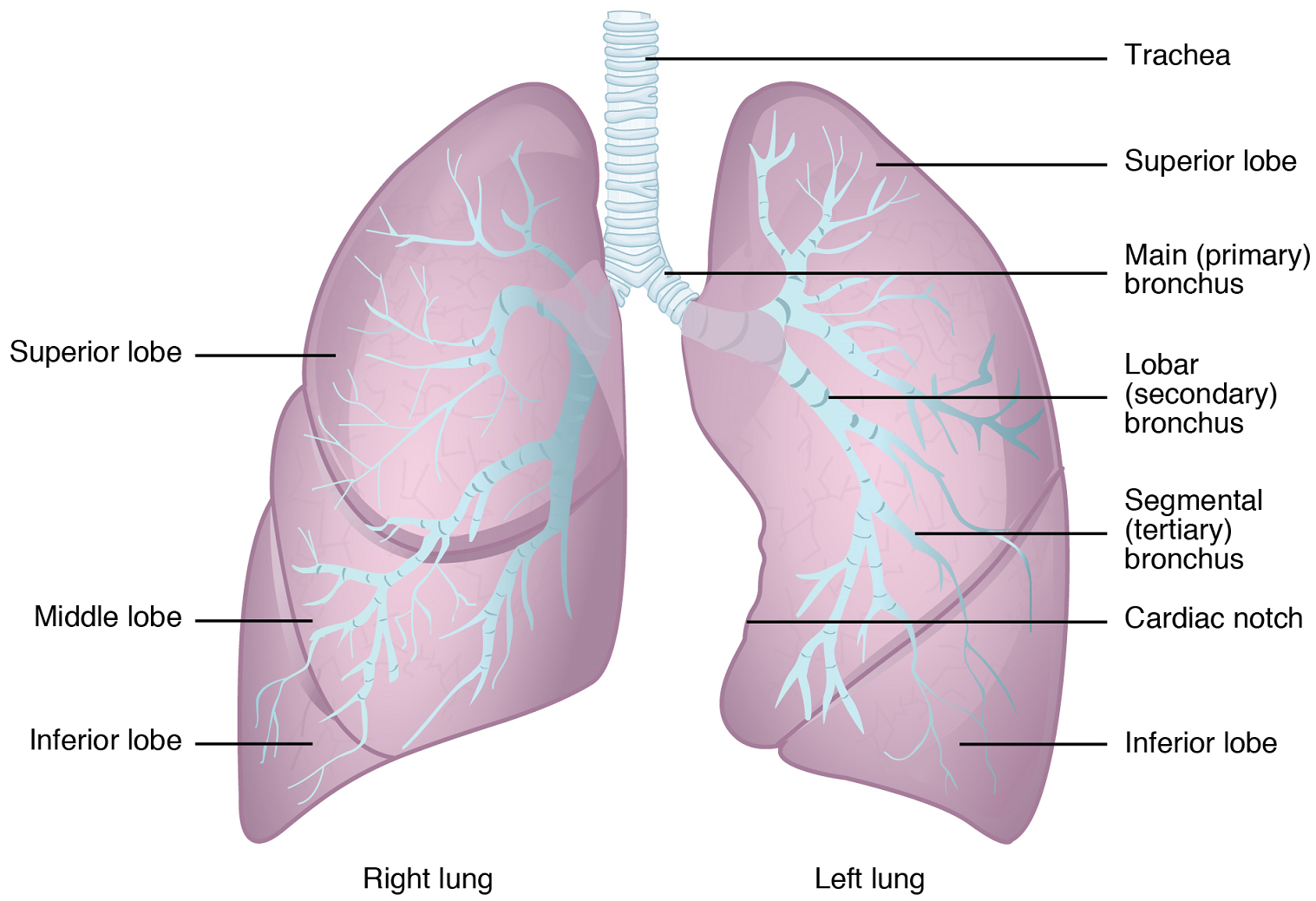
Pleura of the Lungs: Each lung is enclosed within a cavity that is surrounded by a serous membrane called pleura (plural: pleurae). The right and left pleurae enclose the right and left lungs, respectively. The pleurae consist of two layers: the visceral pleura is the layer that is superficial to the lungs (Figure 10). In contrast, the parietal pleura is the outer layer that connects to the thoracic wall and the diaphragm. The visceral and parietal pleurae connect to each other at the hilum (near the branching of the trachea to the primary bronchi). The pleural cavity is the space between the visceral and parietal layers.
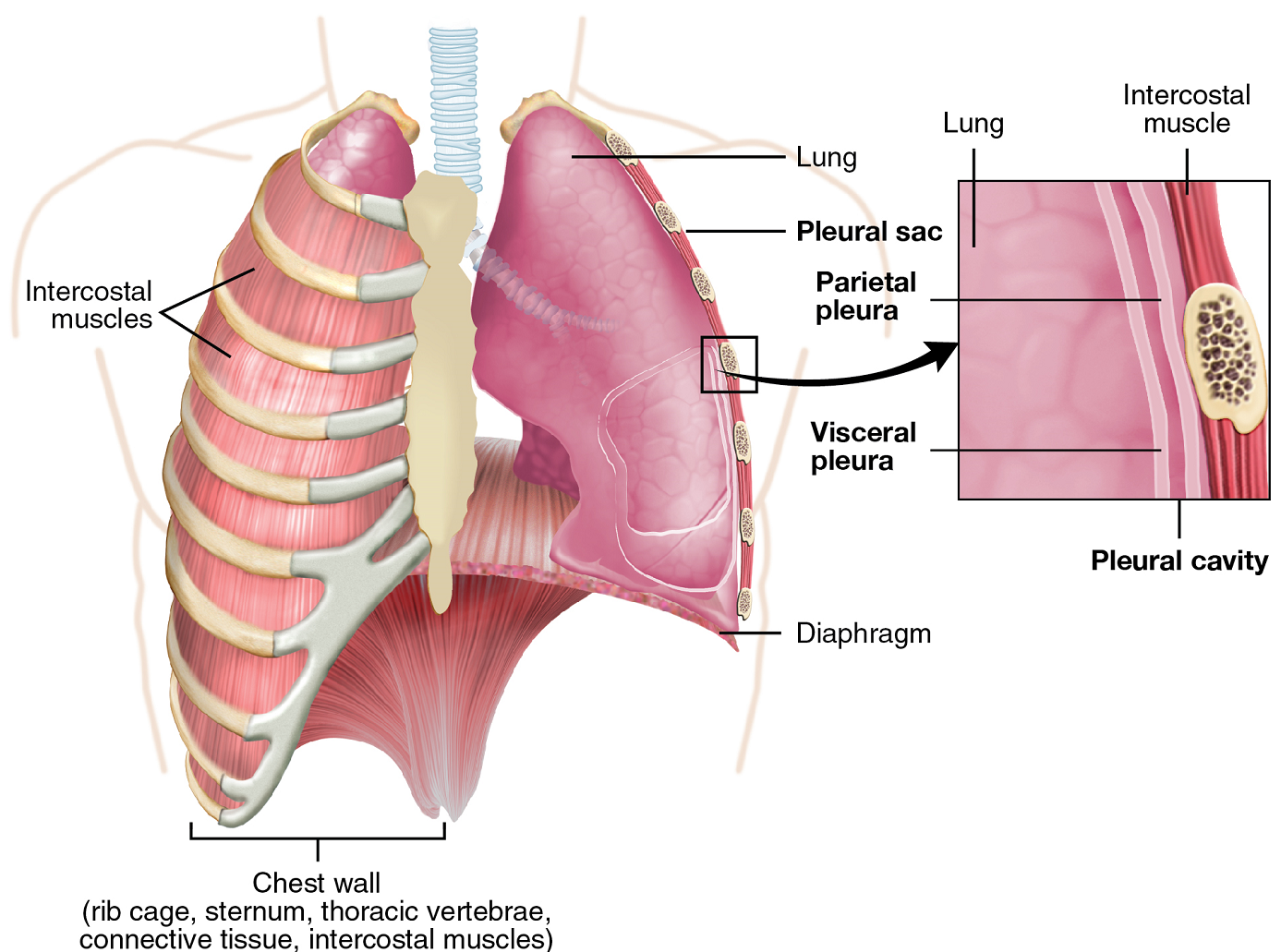
The pleurae perform two major functions: to produce pleural fluid and to create cavities that separate the major organs. Pleural fluid is secreted by cells from both pleural layers into the pleural cavity and acts to lubricate their surfaces. This lubrication reduces friction between the two layers to prevent damage during breathing, and creates surface tension that helps maintain the position of the lungs against the thoracic wall. This adhesive characteristic of the pleural fluid causes the lungs to enlarge when the thoracic wall expands during ventilation, allowing the lungs to fill with air. The division that the pleurae create between major organs prevents interference due to the movement of lungs during breathing and also helps to prevent the spread of infection.
Part 2: The Process of Breathing
Pulmonary ventilation is the act of breathing: the movement of air into the lungs (inspiration or inhalation) and movement of air out of the lungs (expiration or exhalation. The major mechanisms that drive pulmonary ventilation are atmospheric pressure (Patm); the air pressure within the alveoli, called intra-alveolar pressure (Palv); and the pressure within the pleural cavity, called intrapleural pressure (Pip).
Atmospheric pressure is the amount of force that is exerted by gases in the air surrounding any given surface, such as the body. Atmospheric pressure at sea level is 760 mmHg (“millimetres of mercury”). Typically, for respiration, other pressure values are discussed in relation to atmospheric pressure. Therefore, negative pressure is pressure lower than the atmospheric pressure, whereas positive pressure is pressure that is greater than the atmospheric pressure. A pressure that is equal to the atmospheric pressure is expressed as zero.
Intra-alveolar pressure is the pressure of the air within the alveoli, which changes during the different phases of breathing (Figure 12). Because the alveoli are connected to the atmosphere via the tubing of the airways, the intra-alveolar pressure always equalizes with the atmospheric pressure.
Intrapleural pressure is the pressure of the air within the pleural cavity, between the visceral and parietal pleurae. Similar to intra-alveolar pressure, intrapleural pressure also changes during the different phases of breathing. The intrapleural pressure always remains lower than, or negative to, the intra-alveolar pressure (by approximately 4 mmHg).
Understanding Boyle’s Law of gases helps to explain the movement of air in and out of the lungs during pulmonary ventilation. Boyle’s law describes the relationship between volume and pressure in a gas in a confined space, at a constant temperature. Boyle discovered that the pressure of a gas is inversely proportional to its volume: If volume increases, pressure decreases. Likewise, if volume decreases, pressure increases; pressure and volume are inversely related (see Figure 11).
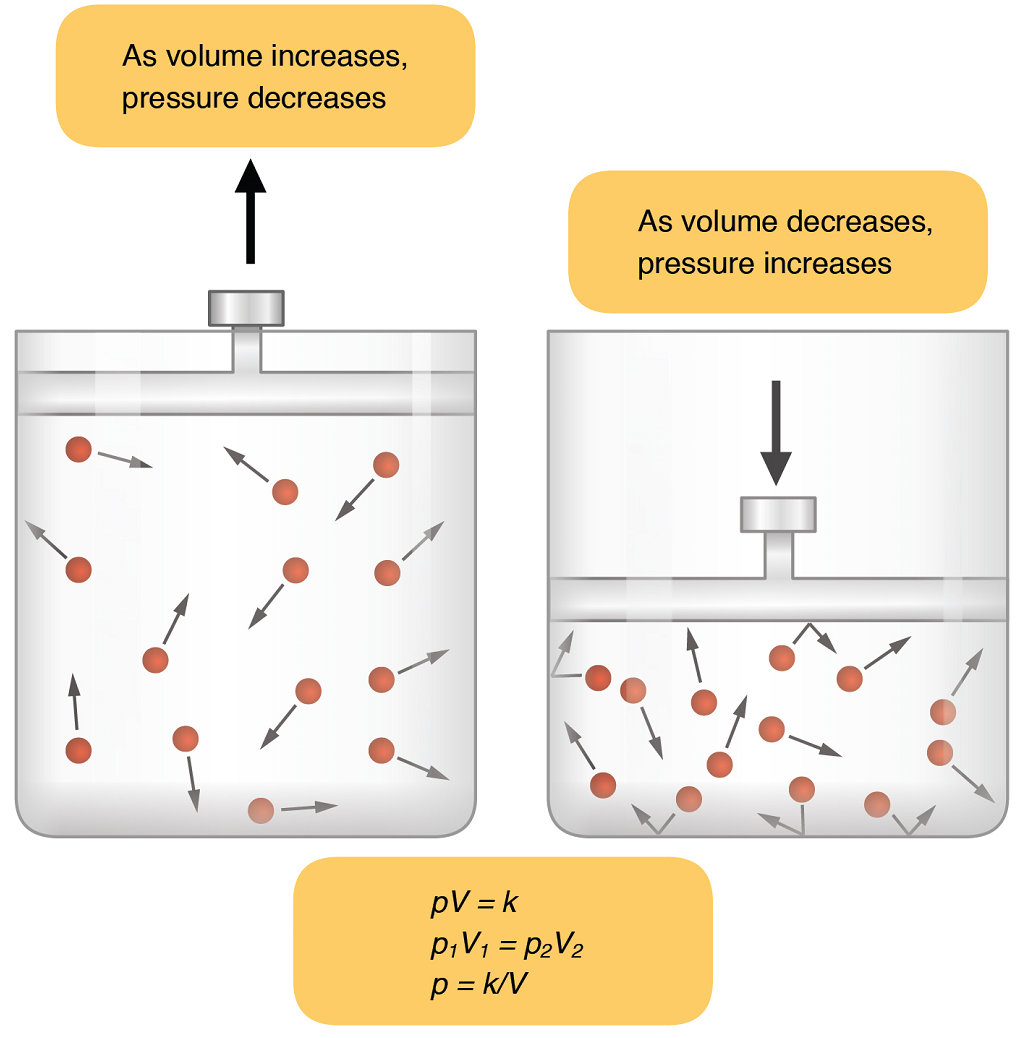
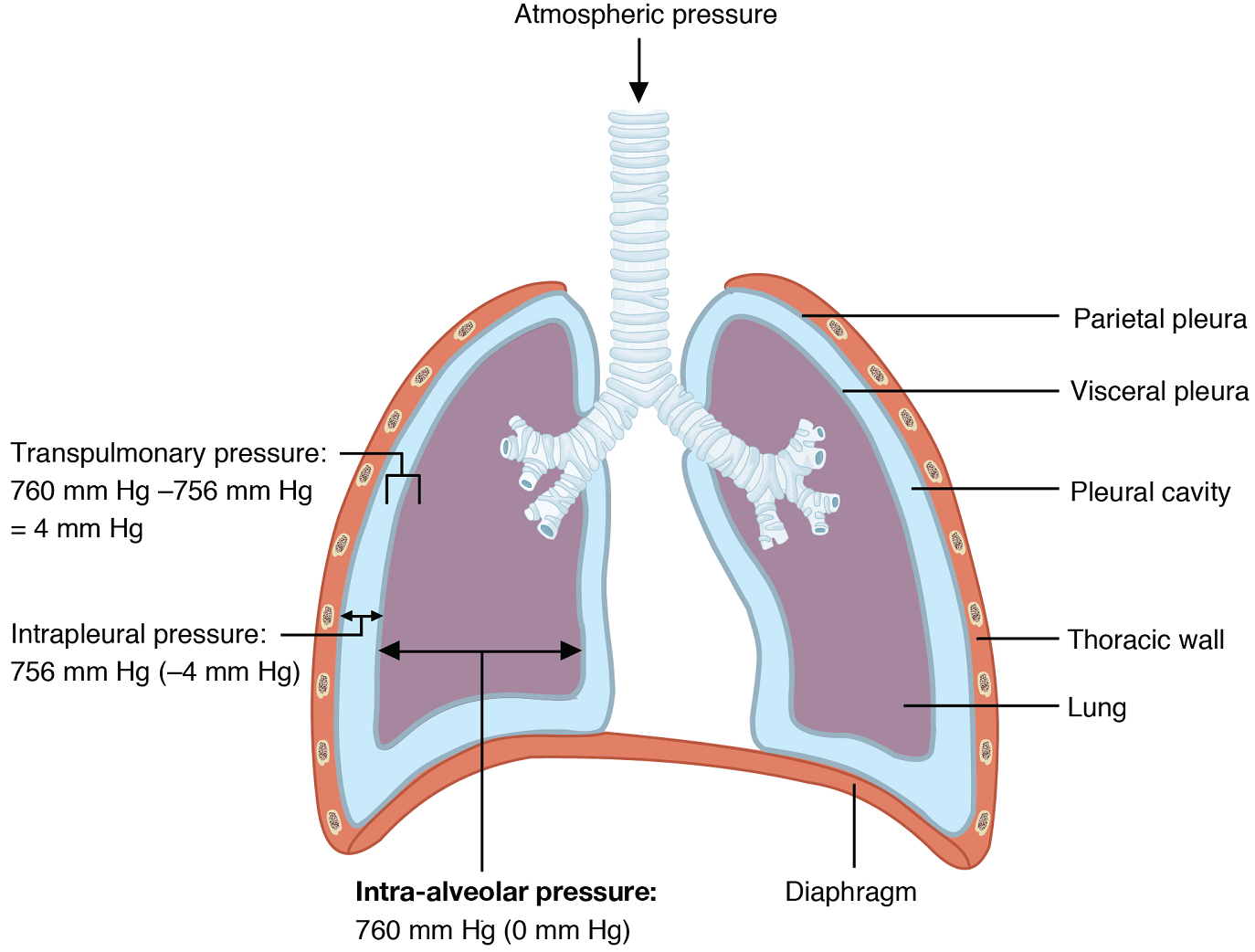
Pulmonary Ventilation: Pulmonary ventilation is mainly dependent on the contraction and relaxation of the diaphragm and the external intercostal muscles which change the intra-alveolar pressure and air flows down a pressure gradient.
A respiratory cycle is one sequence of inspiration and expiration (Figure 13). In general, two muscle groups are used during normal quiet inspiration: the diaphragm and the external intercostal muscles. Additional muscles can be used if a bigger breath is required. When the diaphragm contracts, it moves inferiorly toward the abdominal cavity, creating a larger thoracic cavity and more space for the lungs.

Contraction of the external intercostal muscles moves the ribs upward and outward, causing the rib cage to expand, which increases the volume of the thoracic cavity. Due to the adhesive force of the pleural fluid, the expansion of the thoracic cavity forces the lungs to passively stretch and expand as well. This increase in volume leads to a decrease in intra-alveolar pressure (as stated in Boyle’s Law of Gases), creating a pressure lower than atmospheric pressure. As a result, a pressure gradient is created that drives air into the lungs.
The process of normal expiration is passive, meaning that energy is not required to push air out of the lungs. The diaphragm and external intercostal muscles relax following inspiration and the stretched elastic tissue of the lungs passively recoils. In turn, the thoracic cavity and lungs decrease in volume, causing an increase in intra-alveolar pressure. The intra-alveolar pressure rises above atmospheric pressure, creating a pressure gradient that causes air to leave the lungs.
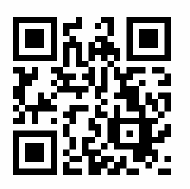
Nervous Control of Ventilation: Breathing usually occurs without thought, although at times you can consciously control it, such as when you swim under water, sing a song, or blow bubbles. The control of ventilation is a complex interplay of multiple regions in the brain, primarily in the brainstem, that signal the muscles used in pulmonary ventilation to contract. The result is typically a rhythmic, consistent ventilation rate that provides the body with sufficient amounts of oxygen, while adequately removing carbon dioxide.
The respiratory rate is the total number of breaths, or respiratory cycles, that occur each minute. Respiratory rate can be an important indicator of disease, as the rate may increase or decrease during an illness or in a disease condition. The respiratory rate is controlled by the respiratory center located within the medulla oblongata in the brain, which responds primarily to changes in carbon dioxide, oxygen, and pH levels in the blood. The normal respiratory rate of a child decreases from birth to adolescence. A child under 1 year of age has a normal respiratory rate between 30 and 60 breaths per minute, but by the time a child is about 10 years old, the normal rate is closer to 18 to 30. By adolescence, the normal respiratory rate is similar to that of adults, 12 to 18 breaths per minute.
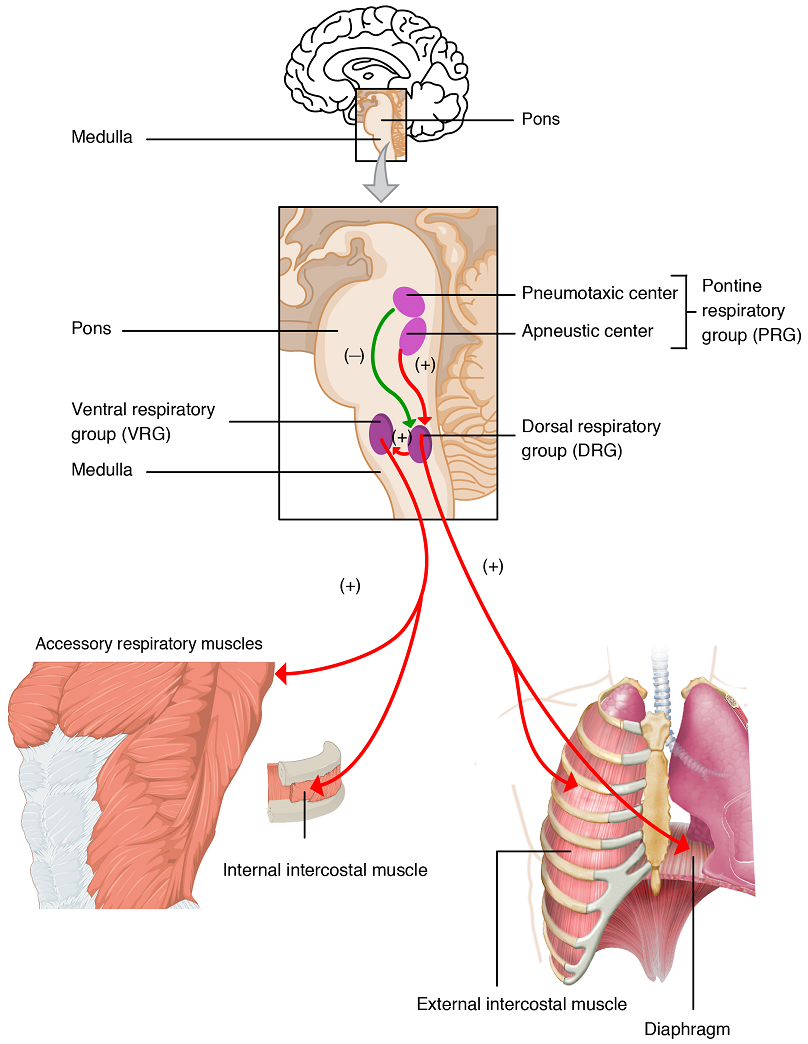
The medulla oblongata includes two main populations of neurons controlling breathing: the dorsal respiratory group (DRG) and the ventral respiratory group (VRG) (Figure 14). These medullary nuclei maintain a constant breathing rhythm by alternatively stimulating the diaphragm and external intercostal muscles to contract, resulting in inspiration, and ceasing this stimulation, resulting in relaxation of these muscle and thus expiration.
Activity of the dorsal and ventral respiratory groups is modulated by input from peripheral stretch and chemoreceptors, and by input from the pontine respiratory group. Neurons in the ventral respiratory group also receive input from the dorsal respiratory group, and can also stimulate the accessory muscles involved in forced breathing to contract, resulting in forced inspiration or forced expiration.
The second respiratory center of the brain is located within the pons, called the pontine respiratory group, and consists of the apneustic and pneumotaxic centers.
The apneustic center is a double cluster of neuronal cell bodies that stimulate neurons in the DRG, controlling the depth of inspiration, particularly for deep breathing. The pneumotaxic center is a network of neurons that inhibits the activity of neurons in the DRG, allowing relaxation after inspiration, and thus controlling the overall rate.
Factors That Affect the Rate and Depth of Respiration: The respiratory rate and the depth of inspiration are regulated by the medulla oblongata and pons; however, these regions of the brain do so in response to systemic stimuli, mainly carbon dioxide (CO2), oxygen (O2) and hydrogen ions (H+) in the blood. Multiple systemic factors are involved in stimulating the brain to produce pulmonary ventilation.
The major factor that stimulates the medulla oblongata and pons to increase respiration is surprisingly not oxygen concentration, but rather the concentration (or partial pressure) of carbon dioxide (CO2) in the blood.
Concentration changes in the blood in certain substances, such as carbon dioxide, oxygen, or hydrogen ions (H+) stimulate chemoreceptors, which in turn signal the respiratory centers of the brain. There are central chemoreceptors located in the brain or brainstem, which are sensitive to are sensitive to hydrogen ions and peripheral chemoreceptors located in two blood vessels: the carotid arteries and aortic arch, which are sensitive to changes in arterial carbon dioxide, oxygen, and hydrogen ion concentrations.
- Central chemoreceptors: As the concentration of carbon dioxide in the blood increases, it readily diffuses across the blood-brain barrier, where it collects in the extracellular fluid. As will be explained in more detail later, carbon dioxide is converted into carbonic acid, which leads to an increased concentration of hydrogen ions (increased acidity or decreased pH). The increase in hydrogen ions in the brain triggers the central chemoreceptors to stimulate the respiratory centers to initiate contraction of the diaphragm and intercostal muscles. As a result, the rate and depth of respiration increase, allowing more carbon dioxide to be expelled, which promotes a reduction in the concentration of carbon dioxide in the blood, and thus hydrogen ions. In contrast, a low concentration of carbon dioxide in the blood causes a low concentration of hydrogen ions in the brain, leading to a decrease in the rate and depth of pulmonary ventilation, producing shallow, slow breathing. Peripheral chemoreceptors are sensitive to arterial carbon dioxide but are less active in stimulating respiratory rate then the central H+ chemoreceptors.
- Peripheral chemoreceptors: Increased hydrogen ion concentration in the blood can be due to increasing carbon dioxide, as mentioned above, but can also be due to other metabolic activities, such as lactic acid accumulation after strenuous exercise or ketoacidosis. Peripheral chemoreceptors of the aortic arch and carotid arteries sense arterial levels of hydrogen ions. When peripheral chemoreceptors sense decreasing, or more acidic, pH levels, they stimulate the respiratory centre and cause an increase in ventilation to remove carbon dioxide from the blood at a quicker rate. Removal of carbon dioxide from the blood helps to reduce hydrogen ions, thus increasing systemic pH.
- Blood oxygen (O2) concentration (or partial pressure of oxygen) is also important in influencing respiratory rate. In addition to their role outlined above, the peripheral chemoreceptors are responsible for sensing large changes in blood oxygen concentration. If blood oxygen concentration falls very low (about 60 mmHg or less) then peripheral chemoreceptors signal the respiratory centre which causes an increase in respiratory activity. The chemoreceptors are only able to sense dissolved oxygen molecules, not the oxygen that is bound to hemoglobin. As will be described in the next section, the majority of oxygen is bound by hemoglobin; when dissolved levels of oxygen drop, hemoglobin releases oxygen. Therefore, a large drop in blood oxygen concentration is required to stimulate the chemoreceptors of the aortic arch and carotid arteries.
- Higher brain centers influence the regulation of breathing by interacting with the respiratory centers. Most of the time, the brainstem regulates breathing involuntarily but we do have some voluntary, conscious control over breathing rate, for example during singing or holding our breath. This is due to signals from the cerebral cortex to the respiratory centers. The hypothalamus and other regions associated with the limbic system are involved in regulating respiration in response to emotions, pain, and temperature. For example, an increase in body temperature or feeling excited (the fight-or-flight response) will result in an increase in respiratory rate.
Respiratory Volumes and Capacities: Respiratory volume is the term used for various volumes of air moved by or associated with the lungs at a given point in the respiratory cycle. There are four major types of respiratory volumes: tidal, residual, inspiratory reserve, and expiratory reserve (Figure 15). Tidal volume (TV) is the amount of air that normally enters the lungs during quiet breathing, which is about 500 milliliters (mL). Expiratory reserve volume (ERV) is the amount of air you can forcefully exhale past a normal tidal expiration, up to 1200 mL for men. Inspiratory reserve volume (IRV) is produced by a deep inhalation, past a tidal inspiration. This is the extra volume that can be brought into the lungs during a forced inspiration. Residual volume (RV) is the amount of air left in the lungs if you exhale as much air as possible. This air prevents alveoli collapsing in on themselves, which makes it possible for alveoli to re-inflate during inhalation. Without residual volume, pulmonary ventilation would cease. Respiratory volume is dependent on a variety of factors, and measuring the different types of respiratory volumes by using a spirometer to generate a spirogram can provide important clues about a person’s respiratory health (Figure 15).
Respiratory capacity is the combination of two or more selected volumes, which further describes the amount of air in the lungs during a given time. For example, total lung capacity (TLC) is the sum of all of the lung volumes (TV + ERV + IRV + RV), which represents the total amount of air a person can hold in the lungs after a forceful inhalation. TLC is about 6000 mL air for men, and about 4200 mL for women. Vital capacity (VC), which is between 4000 and 5000mL, is the amount of air a person can move into or out of his or her lungs, and is the sum of all of the volumes except residual volume (TV + ERV + IRV).
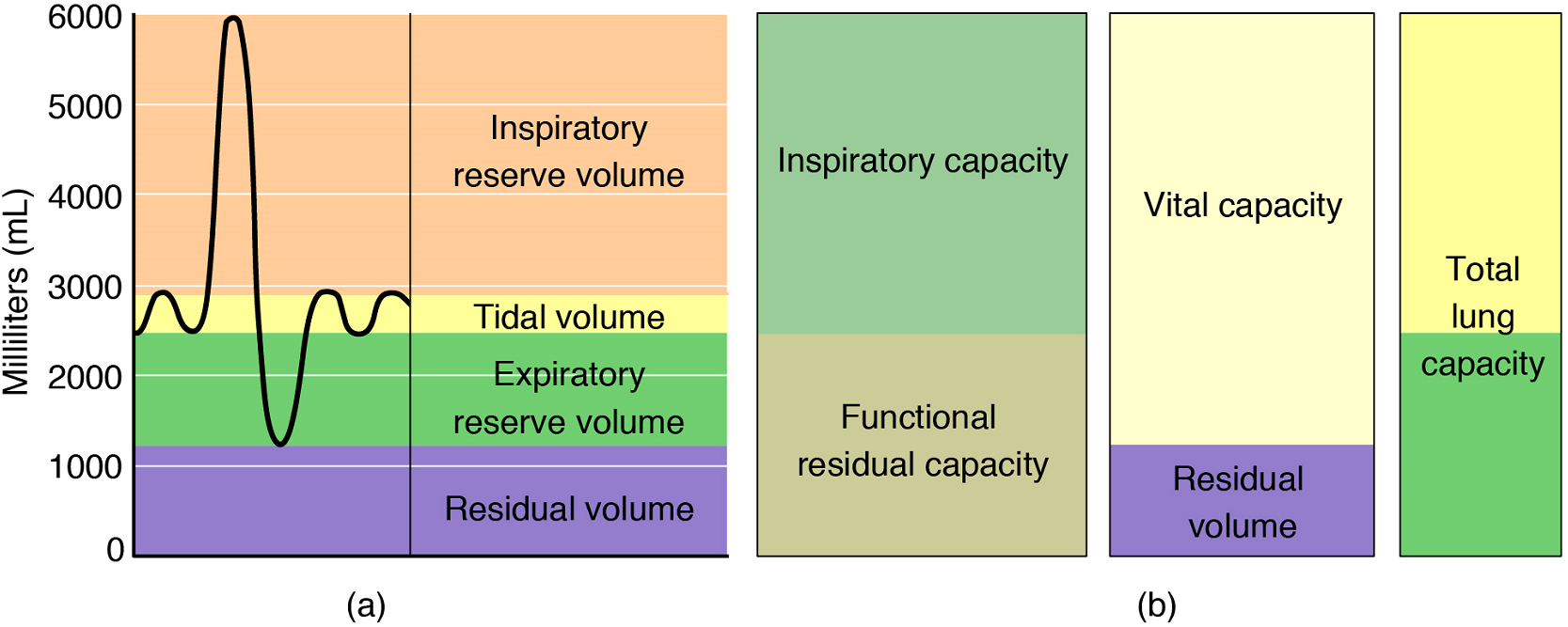
Inspiratory capacity (IC) is the maximum amount of air that can be inhaled past a normal tidal expiration, and is the sum of the tidal volume and inspiratory reserve volume. On the other hand, the functional residual capacity (FRC) is the amount of air that remains in the lung after a normal tidal expiration; it is the sum of expiratory reserve volume and residual volume (see Figure 15).
In addition to the air that creates respiratory volumes, the respiratory system also contains anatomical dead space, which is air that is present in the airway that never reaches the alveoli and therefore never participates in gas exchange. Alveolar dead space involves air found within alveoli that are unable to function, such as those affected by disease or abnormal blood flow. Total dead space is the anatomical dead space and alveolar dead space together, and represents all of the air in the respiratory system that is not being used in the gas exchange process.
Part 3: Gas Exchange
To understand the mechanisms of gas exchange in the lung, it is important to understand the underlying principles of gases and their behavior.
Gas Laws and Air Composition: Gas molecules exert force on the surfaces with which they are in contact; this force is called pressure. In natural systems, gases are normally present as a mixture of different types of molecules. For example, the atmosphere consists of oxygen, nitrogen, carbon dioxide, and other gaseous molecules, and this gaseous mixture exerts a certain pressure referred to as atmospheric pressure (Table 1). Partial pressure (Px) is the pressure of a single type of gas in a mixture of gases (Figure 16). Total pressure is the sum of all the partial pressures of a gaseous mixture; this is Dalton’s law.
Gas | Percent of total composition | Partial pressure (mmHg) |
---|---|---|
Nitrogen (N2) | 78.6% | 597.4 |
Oxygen (O2) | 20.9% | 158.8 |
Water (H2O) | 0.04% | 3.0 |
Carbon dioxide (CO2) | 0.004% | 0.3 |
Others | 0.0006% | 0.5 |
Total | 100% | 760.0 |
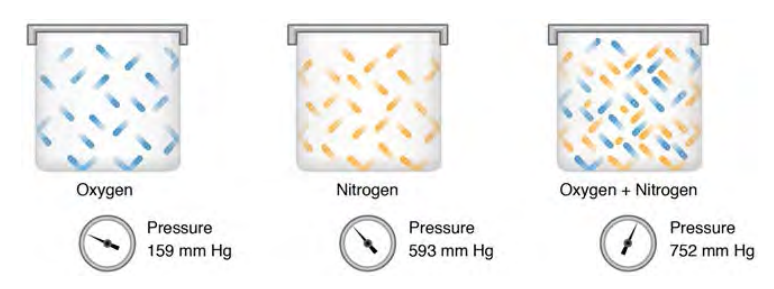
Partial pressure of a gas can be thought of as “concentration” of that gas and, like concentration, partial pressure is extremely important in predicting the movement of gases. A gas will move from an area where its partial pressure is higher to an area where its partial pressure is lower. In addition, the greater the partial pressure difference between the two areas, the more rapid is the movement of gases.
The gases from the atmosphere must be dissolved in water for gas exchange to take place in the alveoli. Atmospheric air dissolves in liquid as it passes through the respiratory system. The partial pressure of a gas and its concentration in a liquid, such as blood, are not identical because solubility of the specific gas plays a factor in concentration. For example, although nitrogen is present in the atmosphere, very little nitrogen dissolves into the blood, because the solubility of nitrogen in alveolar fluid and blood is very low. Oxygen and carbon dioxide are more soluble.
Gas Exchange
Gas exchange occurs at two sites in the body:
- In the lungs, at the respiratory membrane oxygen acquired from inspiration is picked up and carbon dioxide is released and removed via expiration. This exchange of gases with the external environment (atmosphere) in the lungs is known as external respiration.
- At the tissues, oxygen is released into cells and carbon dioxide is picked up from cells. This gas exchange within the internal environment of the tissues of body is known as internal respiration.
The actual exchange of these non-polar, hydrophobic gases occurs due to simple diffusion. Energy is not required to move oxygen or carbon dioxide across membranes. Instead, these gases follow partial pressure gradients that allow them to diffuse.
External Respiration: The pulmonary artery carries deoxygenated blood into the lungs from the heart, where it branches and eventually becomes the capillary network composed of pulmonary capillaries that wrap around the alveoli and form the respiratory membrane (Figure 17). As the blood is pumped through this capillary network, gas exchange occurs.
The anatomy of the lung maximizes the diffusion of gases: The respiratory membrane is highly permeable to gases; the respiratory and blood capillary membranes are very thin; and there is a large surface area throughout the lungs.
Although a small amount of the oxygen is able to dissolve directly into plasma from the alveoli, most of the oxygen is picked up by erythrocytes (red blood cells) and binds to a protein called hemoglobin, a process described later in this chapter. Oxygenated hemoglobin is red, causing the overall appearance of bright red oxygenated blood, which returns to the heart through the pulmonary veins. Carbon dioxide is released in the opposite direction of oxygen, from the blood to the alveoli.

External respiration occurs as a function of partial pressure differences in oxygen and carbon dioxide between the alveoli and the blood in the pulmonary capillaries. Although the solubility of oxygen in blood is not high, there is a drastic difference in the partial pressure of oxygen in the alveoli versus in the blood of the pulmonary capillaries. This large difference in partial pressure creates a very strong pressure gradient that causes oxygen to rapidly cross the respiratory membrane from the alveoli into the blood.
The partial pressure of carbon dioxide is also different between the alveolar air and the blood of the capillary allowing carbon dioxide to diffuse from the blood into the alveoli.
Internal Respiration: Internal respiration is gas exchange that occurs at the level of body tissues (Figure 18). Similar to external respiration, internal respiration also occurs as simple diffusion due to a partial pressure gradient. However, the partial pressure gradients are opposite of those present at the respiratory membrane. The partial pressure of oxygen in tissues is low, because oxygen is continuously used for cellular respiration whereas the partial pressure of oxygen in the blood higher. This creates a pressure gradient that causes oxygen to dissociate from hemoglobin, diffuse out of the blood, cross the interstitial space, and enter the tissue cells. Hemoglobin that has little oxygen bound to it loses much of its brightness, so that blood returning to the heart is more burgundy in color.
Considering that cellular respiration continuously produces carbon dioxide, the partial pressure of carbon dioxide is lower in the blood than it is in the tissue, causing carbon dioxide to diffuse out of the tissue cells, cross the interstitial fluid, and enter the blood. It is then carried back to the lungs either bound to hemoglobin, dissolved in plasma, or in a converted form.
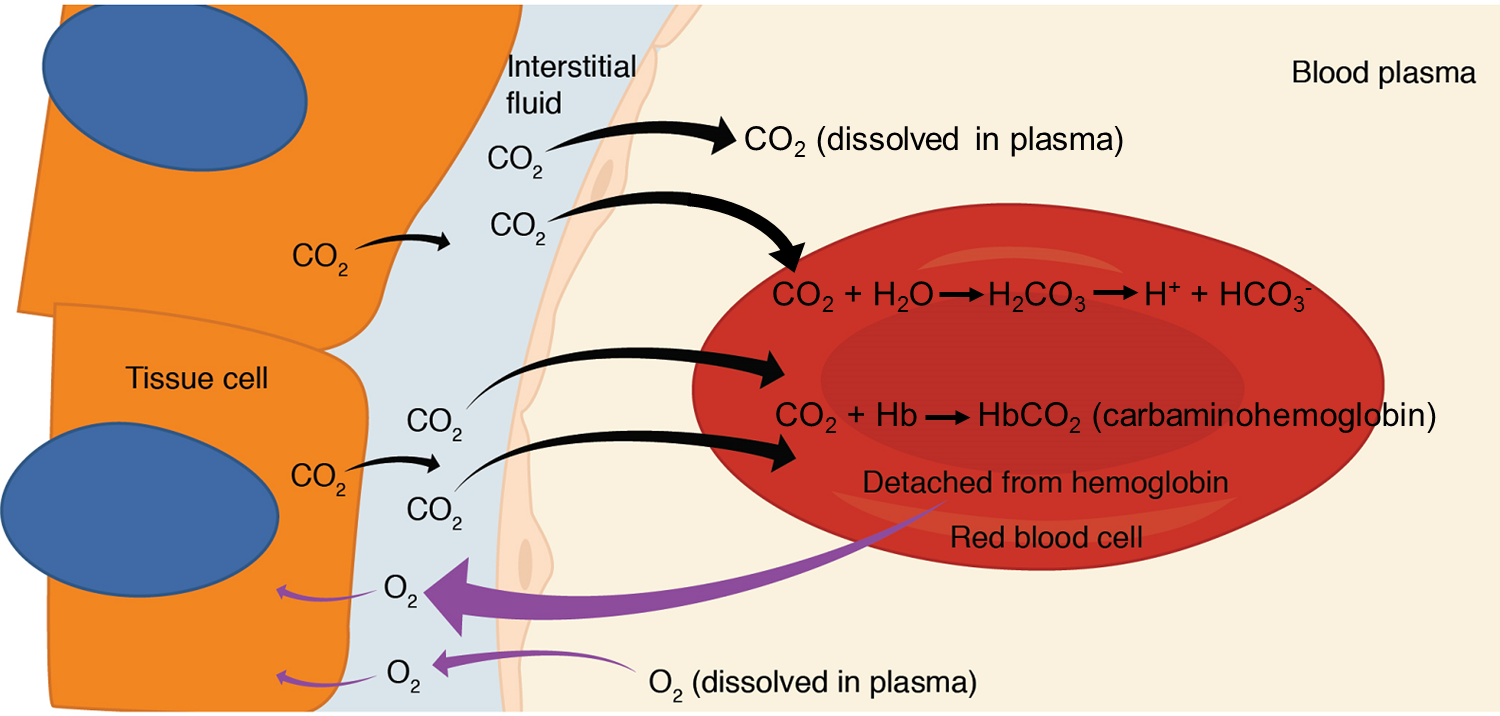
By the time blood returns to the heart, the partial pressure of oxygen has dropped, and the partial pressure of carbon dioxide has returned increased. The blood is then pumped back to the lungs to be oxygenated once again during external respiration.
Part 4: Transport of Gases
The function of respiration is to provide oxygen for use by body cells during cellular respiration and to eliminate carbon dioxide, a waste product of cellular respiration, from the body. In order for the exchange of oxygen and carbon dioxide to occur, both gases must be transported between the external and internal respiration sites. Although carbon dioxide is more soluble than oxygen in blood, both gases require a specialized transport system for the majority of the gas molecules to be moved between the lungs and other tissues.
Oxygen Transport in the Blood: Even though oxygen is transported via the blood, you may recall that oxygen is not very soluble in water. A small amount of oxygen does dissolve in the blood plasma and is transported in the bloodstream, but it is only about 1.5% of the total amount. The majority of oxygen molecules are carried from the lungs to the body’s tissues by a specialized transport system, which relies on the erythrocyte—the red blood cell. Erythrocytes contain a metalloprotein, hemoglobin, which serves to bind oxygen molecules to the erythrocyte (Figure 19). Hemoglobin is composed of four subunits. Each of the four subunits that make up hemoglobin is arranged in a ring-like fashion, with an iron atom covalently bound to the heme in the center of each subunit
Heme is the portion of hemoglobin that binds oxygen. Therefore, one hemoglobin molecule is capable of carrying up to four molecules of oxygen. As oxygen (O2) diffuses across the respiratory membrane from the alveolus to the capillary, it also diffuses into the red blood cell and is bound by hemoglobin (Hb). The following reversible chemical reaction describes the production of the final product, oxyhemoglobin (Hb–O2), which is formed when oxygen binds to hemoglobin. Oxyhemoglobin is a bright red-colored molecule that contributes to the bright red color of oxygenated blood.
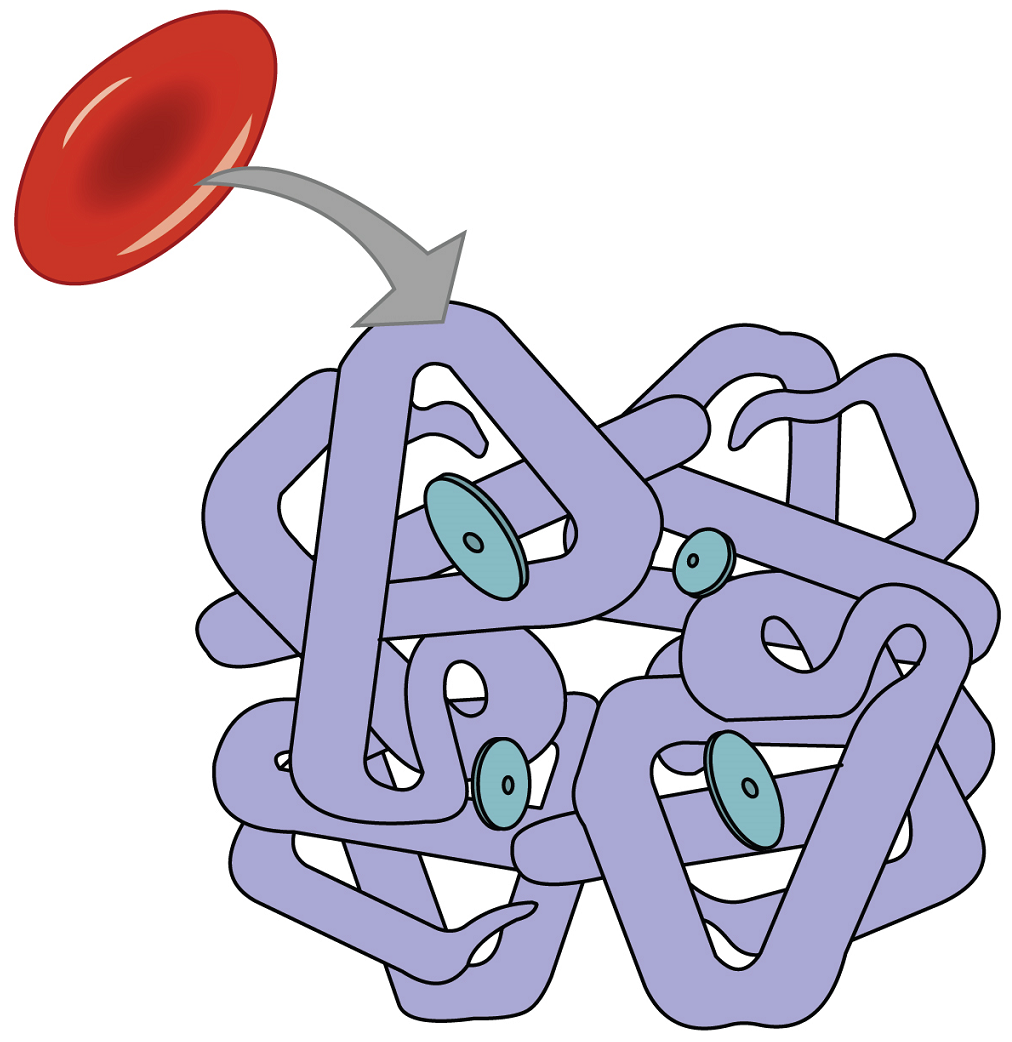
Binding of the first oxygen molecule causes a conformational change in hemoglobin that allows the second molecule of oxygen to bind more readily. As each molecule of oxygen is bound, it further facilitates the binding of the next molecule, until all four heme sites are occupied by oxygen. The opposite occurs as well: After the first oxygen molecule dissociates and is “dropped off” at the tissues, the next oxygen molecule dissociates more readily. When all four heme sites are occupied, the hemoglobin is said to be saturated. When one to three heme sites are occupied, the hemoglobin is said to be partially saturated. Therefore, when considering the blood as a whole, the percent of the available heme units that are bound to oxygen at a given time is called hemoglobin saturation. In a healthy individual with normal hemoglobin levels, hemoglobin saturation generally ranges from 95 percent to 99 percent.
Hb + O2 ↔ HbO2
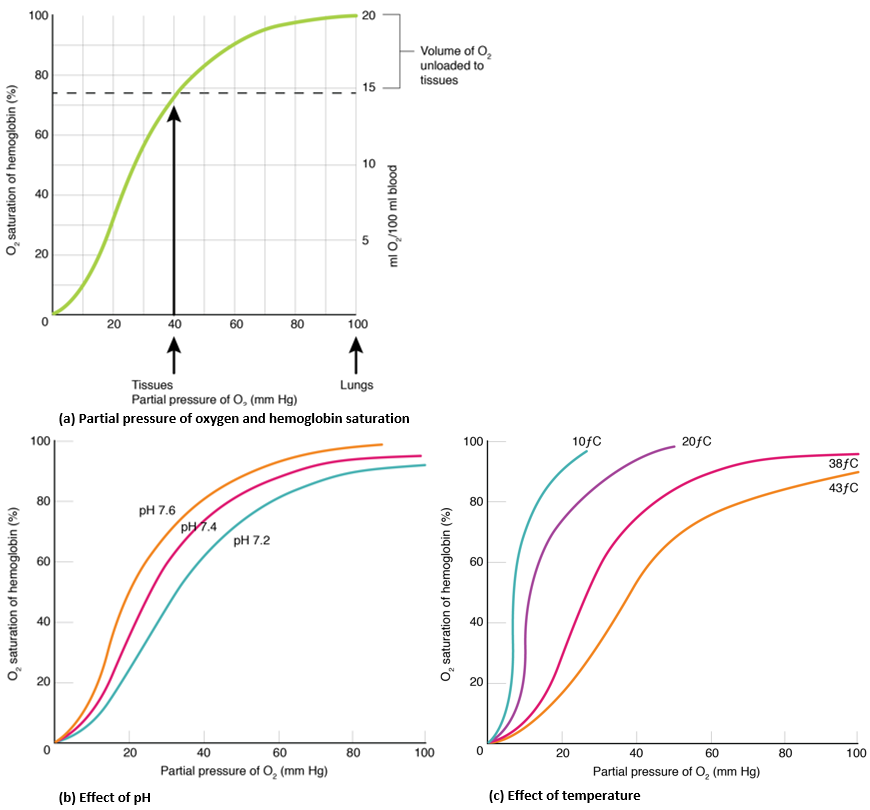
The mechanisms behind the oxygen–hemoglobin saturation/dissociation curve also serve as automatic control mechanisms that regulate how much oxygen is delivered to different tissues throughout the body. This is important because some tissues have a higher metabolic rate than others. Highly active tissues, such as muscle, rapidly use oxygen to produce ATP, lowering the partial pressure of oxygen in the tissue fluid. The difference in partial pressure of oxygen in the muscle tissue and the capillaries becomes quite high. As a result, a greater number of oxygen molecules dissociate from hemoglobin and enter the tissues. The reverse is true of tissues, such as adipose (body fat), which have lower metabolic rates. Because less oxygen is used by these cells, the partial pressure of oxygen within such tissues remains relatively high, resulting in fewer oxygen molecules dissociating from hemoglobin and entering the tissue fluid.
Although venous blood is said to be deoxygenated, some oxygen is still bound to hemoglobin in its red blood cells. This provides an oxygen reserve that can be used when tissues suddenly demand more oxygen.
Factors other than partial pressure of oxygen also affect the oxygen–hemoglobin saturation/dissociation curve. For example, a higher temperature promotes hemoglobin and oxygen to dissociate faster, whereas a lower temperature inhibits dissociation (see Figure 20c). However, the human body tightly regulates temperature, so this factor may not affect gas exchange throughout the body. The exception to this is in highly active tissues, which may release a larger amount of energy than is given off as heat. As a result, oxygen readily dissociates from hemoglobin, which is a mechanism that helps to provide active tissues, such as muscles, with more oxygen.
The pH of the blood is another factor that influences the oxygen–hemoglobin saturation/dissociation curve (see Figure 20b). A lower, more acidic pH promotes oxygen dissociation from hemoglobin. In contrast, a higher, or more basic, pH inhibits oxygen dissociation from hemoglobin. The greater the amount of carbon dioxide in the blood, the more molecules that must be converted to carbonic acid, which in turn generates hydrogen ions and thus lowers blood pH. Furthermore, blood pH may become more acidic when certain byproducts of cell metabolism, such as lactic acid, carbonic acid, and carbon dioxide, are released into the bloodstream. As a result, in metabolically active tissues that create more metabolic acids, oxygen delivery is increased.
Carbon Dioxide Transport in the Blood: Carbon dioxide is transported by three major mechanisms. The first mechanism of carbon dioxide transport is by blood plasma, as some carbon dioxide molecules dissolve in the blood. The second mechanism is transport in the form of bicarbonate (HCO3–), which also dissolves in plasma. The third mechanism of carbon dioxide transport is similar to the transport of oxygen by hemoglobin in erythrocytes (Figure 21).
1. Dissolved Carbon Dioxide: Although carbon dioxide is not considered to be highly soluble in blood, a small fraction—about 7 to 10 percent—of the carbon dioxide that diffuses into the blood from the tissues dissolves in plasma. The dissolved carbon dioxide then travels in the bloodstream and when the blood reaches the pulmonary capillaries, the dissolved carbon dioxide diffuses across the respiratory membrane into the alveoli, where it is then exhaled during pulmonary ventilation.
2. Bicarbonate Buffer: A large fraction—about 70 percent—of the carbon dioxide molecules that diffuse into the blood is transported to the lungs as bicarbonate (HCO3–). Most bicarbonate is produced in erythrocytes after carbon dioxide diffuses into the capillaries, and subsequently into red blood cells. Carbonic anhydrase (CA), an enzyme in red blood cells, causes carbon dioxide (CO2) and water (H2O) to form carbonic acid (H2CO3), which dissociates into two ions: a bicarbonate ion (HCO3–) and a hydrogen ion (H+). The following formula depicts this reaction:
CO2 + H2O ↔ H2CO3 ↔ H+ + HCO3−
Bicarbonate tends to build up in the erythrocytes, so that there is a greater concentration of bicarbonate in the erythrocytes than in the surrounding blood plasma. As a result, some of the bicarbonate will leave the erythrocytes and move down its concentration gradient into the plasma.
At the pulmonary capillaries, the chemical reaction that produced bicarbonate (shown above) is reversed, and carbon dioxide and water are the products. Carbon dioxide diffuses out of the erythrocytes and into the plasma, where it can further diffuse across the respiratory membrane into the alveoli to be exhaled during pulmonary ventilation.
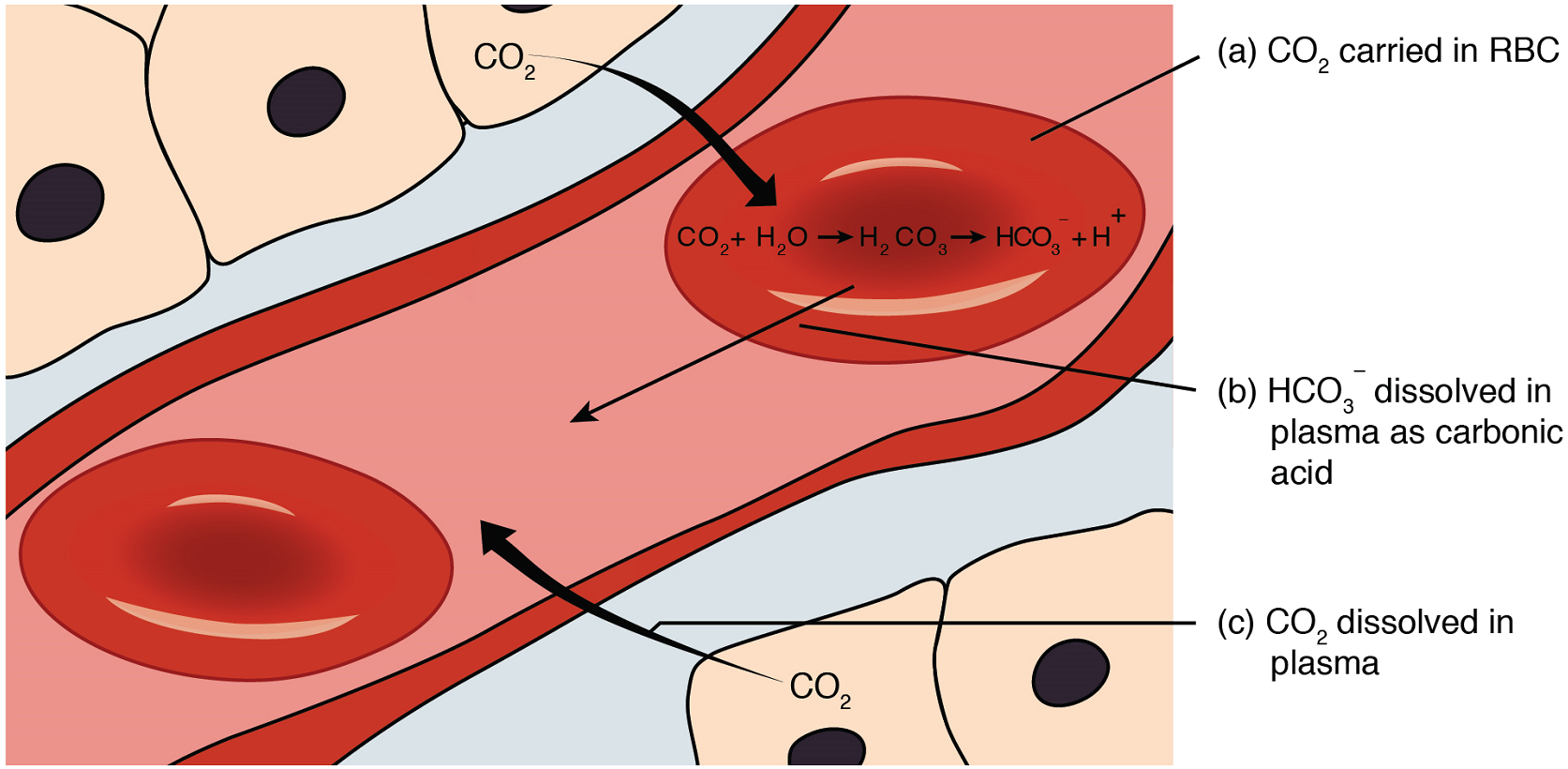
3. Carbaminohemoglobin: About 20 percent of carbon dioxide is bound by hemoglobin and is transported to the lungs. Carbon dioxide does not bind to iron as oxygen does; instead, carbon dioxide (CO2) binds to the amino acid on the protein portions of hemoglobin (Hb) to form carbaminohemoglobin (HbCO2). When hemoglobin is not transporting oxygen, it tends to have a bluish-purple tone to it, creating the darker burgundy color typical of deoxygenated blood. The following formula depicts this reversible reaction:
CO2 + Hb ↔ HbCO2
Similar to the transport of oxygen by heme, the binding and dissociation of carbon dioxide to and from hemoglobin is dependent on the partial pressure of carbon dioxide. Because carbon dioxide is released from the lungs, blood that leaves the lungs and reaches body tissues has a lower partial pressure of carbon dioxide than is found in the tissues.
As a result, carbon dioxide leaves the tissues because of its higher partial pressure, enters the blood, and then moves into red blood cells, binding to hemoglobin. In contrast, in the pulmonary capillaries, the partial pressure of carbon dioxide is high compared to within the alveoli. As a result, carbon dioxide dissociates readily from hemoglobin and diffuses across the respiratory membrane into the air.
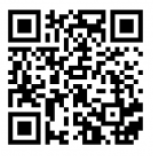
Part 5: Modifications in Respiratory Functions
At rest, the respiratory system performs its functions at a constant, rhythmic pace, as regulated by the respiratory centers of the brain. At this pace, ventilation provides sufficient oxygen to all the tissues of the body. However, there are times that the respiratory system must alter the pace of its functions in order to accommodate the oxygen demands of the body.
Hyperpnea: Hyperpnea is an increased depth and rate of ventilation to meet an increase in oxygen demand as might be seen in exercise or disease, particularly diseases that target the respiratory or digestive tracts. This does not significantly alter blood oxygen or carbon dioxide levels, but merely increases the depth and rate of ventilation to meet the demand of the cells. In contrast, hyperventilation is an increased ventilation rate that is independent of the cellular oxygen needs and leads to abnormally low blood carbon dioxide levels and high (alkaline) blood pH.
Hyperventilation can occur for a multitude of reasons. It may be caused by abnormal functioning of the lungs, as a result of conditions such as asthma or early emphysema. It can occur at high altitudes where partial pressure of oxygen decreases (Table 2) and leads to lower hemoglobin saturation in the blood; hemoglobin saturation is about 67 percent at 19,000 feet above sea level, whereas it reaches about 98 percent at sea level. Finally, hyperventilation may be caused by increased metabolism as a result of such conditions as hyperthyroidism, infection, or fever.
Example location | Altitude (km above sea level) | Atmospheric pressure (mmHg) | Partial pressure of oxygen (mmHg) |
---|---|---|---|
Vancouver, British Columbia | 0-0.5 km | 707-760 | 148-159 |
Lake Louise, Alberta | 1.6 km | 638 | 133 |
Aspen, Colorado | 2.4 km | 565 | 118 |
Mount Logan, Yukon | 6.0 km | 324 | 67 |
Mount Everest, Tibet | 8.8 km | 260 | 54 |
Although it has no effect on oxygen levels in the blood, hyperventilation significantly reduces the amount of carbon dioxide in the blood. This reduction in carbon dioxide levels in turn leads to reduced carbonic acid levels in the blood, which results in alkalosis (blood plasma pH higher than normal). Decreased blood carbon dioxide also decreases blood pressure, as the signals coming from peripheral carbon dioxide receptors (normally stimulated by CO2) decrease in frequency and cause the vasomotor center in the medulla oblongata to reduce constriction of the smooth muscle in the walls of blood vessels and allow vasodilation. Finally, low carbon dioxide and the associated high pH interfere with the ability of hemoglobin to release oxygen molecules to body tissues, including the brain, which can cause dizziness or unconsciousness.
Hypoxia: Hypoxia is a reduction in the amount of oxygen reaching body tissues. It may be caused by a deficiency in atmospheric oxygen, whether due to high altitude (as described above) or being in an enclosed space with limited airflow (e.g. a crowded room with poor ventilation).
Hypoxia may also be caused by physiological problems with the respiratory or cardiovascular system. In the case of the respiratory system, any interference in the process of breathing (e.g. abnormal muscle contractions) or obstruction in the air passages (e.g. excessive mucus) will cause hypoxia by ventilatory deficiency. Alternatively, hypoxia may be caused by a pulmonary diffusion defect in which the diffusion of oxygen gas across the respiratory membrane is impaired. Fluid in the pulmonary alveoli, for example, increases the distance across which oxygen must diffuse through liquid, effectively increasing the thickness of the respiratory membrane and therefore slowing the rate at which oxygen can move into the blood. In the cardiovascular system, hypoxia may be caused by a hemoglobin deficiency or a circulatory deficiency. A hemoglobin deficiency may be the result of anemia, where there is a shortage of functional red blood cells. It may also be the result of conditions such as carbon monoxide poisoning, where carbon monoxide displaces oxygen bound to hemoglobin molecules, rendering the hemoglobin incapable of carrying oxygen and thus effectively nonfunctional. Circulatory deficiencies may be the result of obstruction of a blood vessel (e.g. as a result of atherosclerosis), of low blood pressure (hypotension), or of structural problems that make the cardiovascular system less efficient than it should normally be (e.g. if the ductus arteriosus or foramen ovale fail to close after birth).
Finally, hypoxia may result from edema, where excessive fluid accumulates around cells, for example as a result of inflammation, renal failure, or congestive heart failure. This fluid buildup may occur in the lung tissue or alveoli (pulmonary edema), where it slows the diffusion of oxygen across respiratory membranes, or in other tissues where it can slow the diffusion of oxygen to body cells.
Hypoxia can result in cyanosis, where the skin and mucous membranes take on a bluish (or purplish) discoloration. It can also result in tachycardia, or increased heart rate, and dizziness as a result of insufficient oxygen reaching the brain.